MASS PROPERTIES OF DEEP-SEA SEDIMENTS
Color of Deep-sea Sediments. The significance of color has been considered above, and in the system of classification it was pointed out that pelagic deposits are characterized by light colors or red or brown
The colors of the various types of pelagic sediments are as follows:
Globigerina ooze. Milky white, rose, yellow, or brown. Near land it may be dirty white, gray, or blue.
Pteropod ooze. White to light brown with reddish, pink, or yellow tinge.
Diatom ooze. Yellowish, straw, or cream colored. Dirty white when dry. Darker near land.
Radiolarian ooze. Red, chocolate brown, or straw colored.
Red clay. In North Atlantic, brick red, but in South Pacific and Indian Oceans, chocolate brown. Graduates into “blue” near shore.
As pointed out in the section on classification, the color of terrigenous deposits is extremely variable. Murray and his co-workers established various types of terrigenous sediments to which the names “blue,” “green,” and “red” muds were applied. Unfortunately, the color was not the only factor which placed a given sample within one of these groups, therefore the anomalous situation developed that a green- or brown-colored mud would be classified as a “blue mud.”
Texture of Deep-sea Sediments. The texture of pelagic sediments varies a great deal, depending largely upon the proportions of organic remains. Pteropod and globigerina oozes, containing little or no fine material, which occur on topographic highs actually are sands if texture alone is considered. At the other extreme are the fine-grained clays. It is convenient, as in the discussion of color, to consider the textural properties of the three extreme types of pelagic sediments, namely, red clay, diatom ooze high in organic remains, and globigerina ooze largely made up of foraminiferal skeletons. Intermediate types representing organic oozes diluted with clay will of course fall within the limits described by these extreme types. Coarse-grained mineral fragments are never abundant except under special circumstances.
The following data have been selected as examples. The average of five globigerina oozes from the Pacific Ocean collected by the Carnegie were analyzed by the pipette method by Revelle (1936). These samples
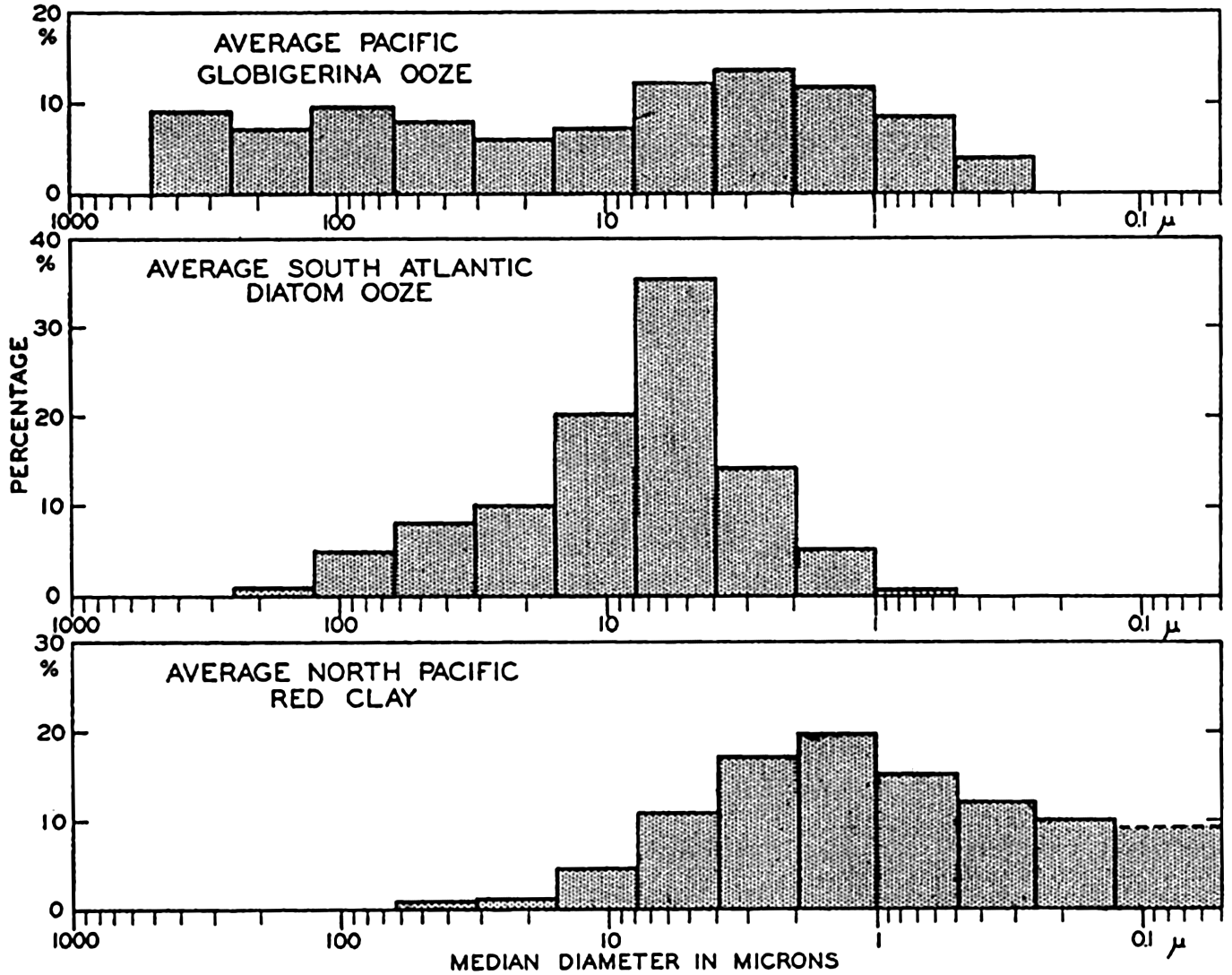
Distribution of particle size (equivalent diameter) in pelagic sediments represented by histograms (same data as in fig. 255).
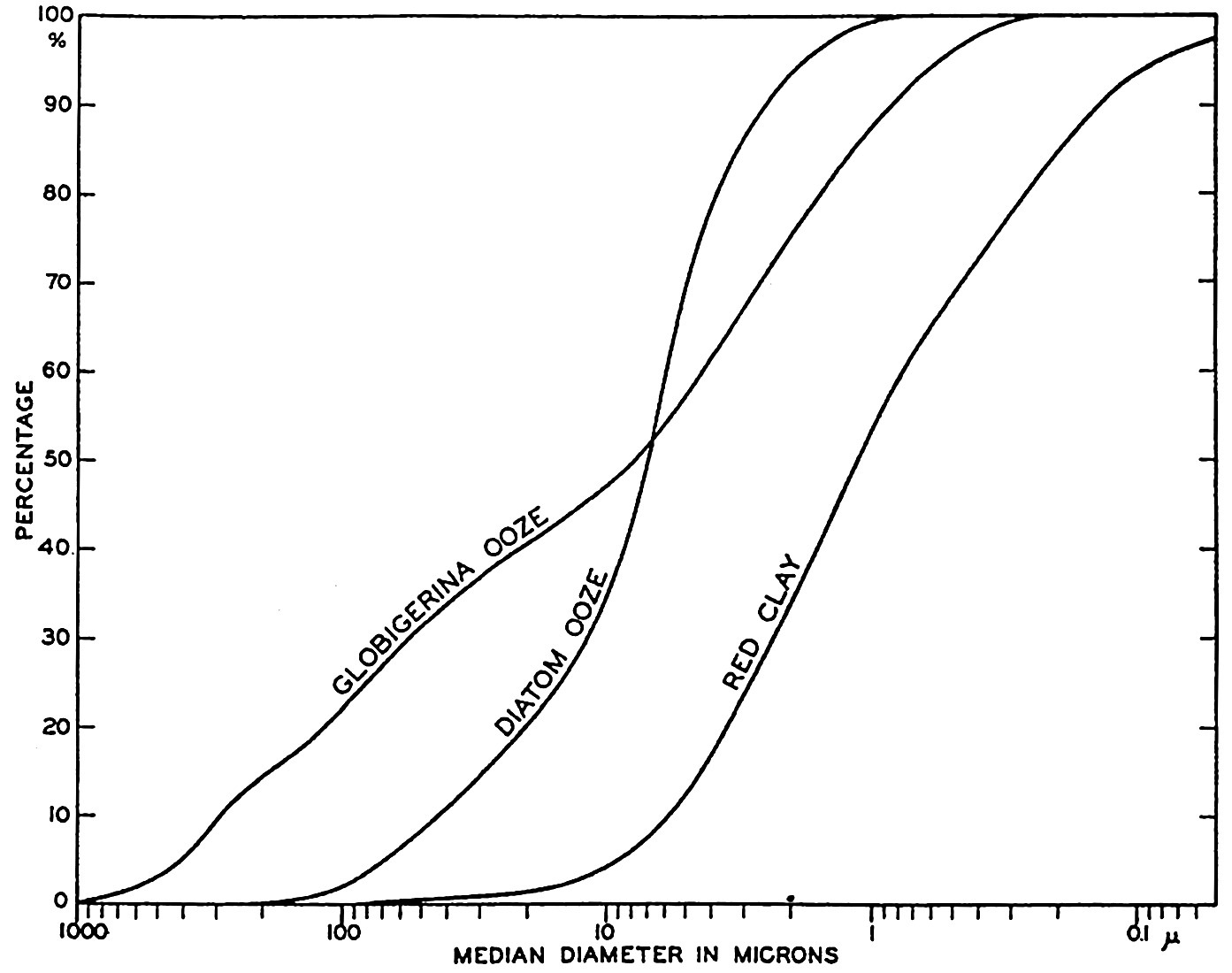
Distribution of particle size (equivalent diameter) in pelagic sediments represented by histograms (same data as in fig. 254).
Sediment | Sand % | Silt % | Clay % | M2 μ | Q1 μ | Q3 μ | So | Sk |
---|---|---|---|---|---|---|---|---|
Globigerina ooze | 28.7 | 33.5 | 37.8 | 7.8 | 2.0 | 82.0 | 6.4 | 4.58 |
Diatom ooze | 6.0 | 88.0 | 6.0 | 7.0 | 4.4 | 15.0 | 1.85 | 3.63 |
Red clay | 0.0 | 17.3 | 82.7 | 1.1 | 0.35 | 2.8 | 2.83 | 0.94 |
The median diameter for the globigerina ooze does not represent the size of the complete tests which would mostly have diameters greater than 62.5 microns. It will be noted that the histogram shows several maxima. The histograms of globigerina oozes show two maxima characteristically, one in the coarser grades which corresponds to the relatively complete tests, and another in the fine grades. Revelle (1936) has shown that corresponding maxima are found in the CaCO3 content of the different size grades. The maximum in the small grades is due to the presence of small crystals of CaCO3 (calcite) which are believed to arise from the mechanical disintegration of the foraminiferal tests. Correns (1939) considers that the fine-grained calcareous particles result from the destruction of the albuminoid (pseudochitin) cement which binds together the carbonate crystals in the shells. More than 50 per cent of the material finer than 2 microns may be calcareous. The data for the diatom ooze show that the bulk of the material is in the silt grades with the largest fraction between 7.8 and 3.9 microns. Because of their shape and structure, radiolarian and diatom frustules will have equivalent diameters smaller than their true dimensions. Revelle (1936) shows in photomicrographs many entire radiolarians of dimensions larger than 250 microns, and the material between 250 and 125 microns is almost entirely composed of diatom frustules and smaller radiolarians.
From the foregoing discussion it may be seen that the pelagic deposits contain material of diameters ranging from a millimeter or more down to the clay fraction with diameters chiefly less than one micron. Excluding the more or less exotic volcanic material and such rare constituents as manganese nodules, sharks’ teeth, and so forth, it is obvious that the coarser fractions of true pelagic deposits are entirely composed of skeletal remains, whereas at the other extreme there is the inorganic clay fraction. The size-grade composition of a given sample may fall anywhere between these extremes and have only one maximum or several maxima, depending upon the character of the source materials and the relative proportions in which they are deposited.
Physical Composition of Deep-sea Sediments. The character of the grains larger than about two microns can generally be determined by microscopic examination. The organic remains can be identified, and with the aid of the microscope and various techniques of the petrographer the mineral grains may also be recognized. The fine material, such as that predominating in red clays, cannot be studied in this way because of the small size of the individual particles. For many years this material was considered as “amorphous,” but the development of X-ray methods for determining crystal structure and the application of various physical and chemical tests have established the identity of the clay minerals.
In the examination of sediment samples the mechanical analysis is usually made first and various convenient size grades are separated.
Organic constitutents in deep-sea sediments comprise remains of virtually every type of marine organisms possessing hard skeletal structures. The various oozes may consist almost entirely of the remains of one type of planktonic organisms; on the other hand virtually all types of organisms may be found in any one sample, although the total may not make up more than a small percentage of the deposit. The latter situation may prevail in red clays. Foraminifera, both planktonic and benthic, have been studied most extensively, and the species encountered and their distribution are fairly well known. Much less information is available concerning the species of diatoms, radiolarians, and other forms encountered in marine deposits. In many reports on the physical composition of marine sediments no attempt has been made to classify the organic remains beyond reporting the percentages of calcareous and siliceous structures. Revelle (1936) has tabulated the general organic constituents in 1156 pelagic sediment samples studied by Sir John Murray and his associates. These data are given in table 108, taken from Revelle. In it are shown the maximum, minimum, and average percentages of calcium carbonate, planktonic and benthic foraminifera, other calcareous remains, siliceous remains, and the mineral grains of diameters greater and less than 0.05 mm (50 microns) in the various types of pelagic sediments. If the extreme cases are selected, as in the case of the texture, it may be seen that red clays may contain 100 per cent of minerals less than 0.05 mm, whereas calcareous oozes may contain more than 98 per cent of calcareous remains, and siliceous oozes 90 per cent of siliceous remains. However, most samples represent mixtures of two or more of these basic types of constituents in variable proportions. The average percentage of various organic and inorganic materials can be obtained from table 108.
Calcareous structures are in general more abundant than siliceous organic remains. The various groups of pelagic and benthic organisms which may contribute to the sediments have been listed elsewhere (p. 950). Both planktonic and benthic foraminifera are found in marine sediments. Cushman (1928) lists 25 species of planktonic foraminifera. Revelle found 23 species in the deposits of the Pacific Ocean, Throp (1931) 19 species in the deposits of the western North Atlantic, and W. Schott (in Correns et al, 1937) 21 species in the Equatorial Atlantic.
Physical composition | Red clay (%) | Radiolarian ooze (%) | Diatom ooze (%) | Globigerina ooze (%) | Pteropod ooze (%) | ||||
---|---|---|---|---|---|---|---|---|---|
(C) | (M) | (C) | (C) | (V) | (C) | (M) | (M) | ||
a Only in two exceptional cases; the usual maximum is not more than 5 per cent. | |||||||||
b Only in one exceptional case. | |||||||||
c Includes finely divided remains of siliceous organisms. | |||||||||
CaCO3 | Maximum | 28.8 | 29.0 | 20.0 | 36.3 | 24.0 | 96.8 | 97.2 | 98.5 |
Minimum | 0 | 0 | tr | 2.0 | 0 | 80.2 | 30.0 | 44.8 | |
Average | 6.7 | 10.4 | 4.0 | 23.0 | 2.7 | 64.5 | 64.7 | 73.9 | |
Planktonic foraminifera | Maximum | 27.0 | predominant part of CaCO3 | 80.0 | 95.0 | 75.0 | |||
Minimum | 0 | 25.0 | 15.0 | 15.0 | |||||
Average | 4.77 | 8.8 | 3.1 | 3.1 | 53.1 | 58.9 | 34.7 | ||
Benthic foraminifera | Maximum | 3.0 | 10.0 | 10.0 | |||||
Minimum | 0 | present | 0 | tr | |||||
Average | 0.6 | 0.6 | .1 | 1.6 | 2.1 | 2.1 | 3.6 | ||
Other calcareous remains | Maximum | 6.3 | 31.8 | 26.0 | 57.0 | ||||
Minimum | 0 | present | 1.2 | tr | 15.8 | ||||
Average | 1.3 | 1.0 | .8 | 3.2 | 9.2 | 3.7 | 35.5 | ||
Siliceous remains | Maximum | 5.0 | 80.0 | 60.0 | 90.0 | 10.0 | 15.0[a] | 20.0 | |
Minimum | 0 | 30.0 | 20.0 | 40.0 | 4.0 | tr | tr | ||
Average | 2.4 | 0.7 | 54.4 | 41.0 | 73.1 | 1.6 | 1.7 | 1.9 | |
Texture of mineral particles | |||||||||
>.05 mm, diameter | Maximum | 20.0 | 60.0[b] | 5.0 | 25.0 | 40.0 | 50.0[b] | 50.0[b] | 20.0 |
Minimum | 1.0 | tr | 1.0 | 3.0 | 1.0 | 1.0 | tr | tr | |
Average | 5.6 | 2.4 | 1.7 | 15.6 | 8.4 | 5.3 | 5.1 | 4.7 | |
<.05 mm, diameter | Maximum | 100.0 | 67.0[c] | 27.9[c] | 34.0 | 66.6 | 69.3 | 41.8 | |
Minimum | 31.0 | 17.0[c] | 12.5[c] | 9.0 | 1.2 | 1.2 | tr | ||
Average | 85.4 | 86.5 | 39.9[c] | 20.4[c] | 15.8 | 30.6 | 28.5 | 19.6 | |
Number of samples averaged | 70 | 126 | 9 | 5 | 16 | 118 | 772 | 40 |
According to the studies of W. Schott (1937) the benthic foraminifera constitute less than 1 per cent of the total number of foraminifera in about 50 per cent of the samples examined. In very few cases do they exceed 25 per cent of the total number of tests. The highest percentages occur in samples of intermediate CaCO3 content. This is in agreement with Revelle (1936), who found the greatest number of species in samples of intermediate carbonate content.
W. Schott (1937) has introduced the “foraminiferal number” as a measure of the abundance of relatively entire tests. He found that most of the unbroken tests were in the size grade between 2 to 0.2 mm diameter. By count he determined that 1 g of this material contained 23,300 tests. The foraminiferal number is then obtained by multiplying the fraction of the material in this size grade, less the percentage of material other than foraminiferal tests, by 23,300. From this it can be seen that a sample composed entirely of foraminifera in this grade would have a foraminiferal number of 23,300. The highest value obtained by Schott was 12,700. Much of the calcium carbonate is present as smaller tests or as broken fragments, hence the foraminiferal number is not directly proportional to the CaCO3. The relationship obtained by Schott is shown in fig. 256. Correns (1939) has suggested that the term globigerina ooze be restricted to samples with foraminiferal numbers greater than 6000, which would indicate that at least one quarter of the sediment consisted of unbroken foraminiferal tests in the size grade of diameter between 2 and 0.2 mm. As can be seen from fig. 256, such samples would contain more than 60 per cent CaCO3.
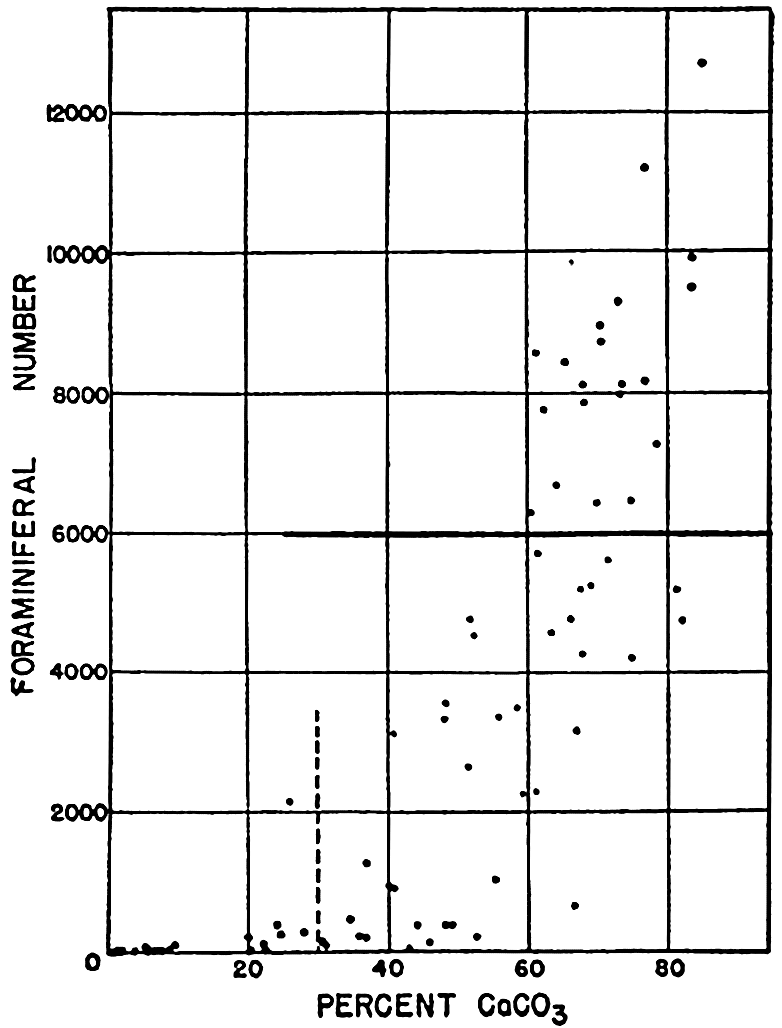
Relation of the foraminiferal number to the calcium carbonate content in sediments from the Equatorial Atlantic Ocean. (From Correns, 1939, in Recent Marine Sediments, edited by Parker D. Trask, and published by the American Association of Petroleum Geologists, Tulsa, Oklahoma.)
Because of their relatively large size, pteropods and heteropods are conspicuous constituents of certain calcareous oozes from the shallower depths in tropical latitudes. However, they never constitute more than
Calcareous remains of other types of pelagic and benthic animals may be found in pelagic deposits but they are never abundant. Echinoderms, annelids, ostracods, and polyzoa are among the benthic forms commonly present.
Calcareous structures of plant origin are among the important constituents of the pelagic deposits. Fragments of the larger calcareous algae (Lithothamnion and Corallina) are often found near land, and in the open ocean the remains of the microscopic Coccolithophoridae may be very abundant, particularly in the tropical and subtropical parts of the Atlantic Ocean. These pelagic forms are so delicate that the entire skeletons are rarely found but, instead, only the tiny plates of which they are built up. The oval plates called coccoliths and the rod-shaped fragments called rhabdoliths, which arise from the coccosphere and the rhabdosphere, may constitute as much as 74 per cent of the material between 10 and 2 microns in diameter and may form about 15 per cent of the material of finer grades. According to Correns (1939), the calcareous plant remains never form more than 13 per cent of the entire sample.
The inorganic constituents in deep-sea sediments are shown in table 109 (Revelle, 1936), giving the frequency with which the principal minerals were reported by Murray and his co-workers in the various types of pelagic deposits. These records are based on examination of the material larger than 0.05 mm and do not include the smaller particles referred to by Murray as “fine washings.” Table 108 gives the maximum, minimum, and average content of “minerals” and “fine washings” in the various types of deposits and, as can be seen from the average values, the larger grains rarely represent more than a small percentage of a pelagic sediment. Coarse terrigenous material must be rare except in regions where ice or the atmosphere are effective, because of the lack of adequate transporting agencies. Volcanic material, except pumice which will float for some time and the fine dust which may be air-borne, will also be restricted to areas of volcanic activity. The authigenic materials, on the other hand, are not directly dependent upon transporting agencies but are related to the environment prevailing in and over the sediment.
Although means have been available for many years for the identification of mineral grains, it is only recently that it has been possible to obtain any adequate idea as to the character of the fine material. Murray considered it as amorphous, that is, noncrystalline, and from the available data decided that red clays and the clay fraction of all pelagic deposits were formed on the sea bottom by the decomposition of volcanic material. It was also suggested that a certain “insoluble” fraction of calcareous and siliceous skeletons might contribute to this fraction of the deposit.
Percent of samples analyzed in which sustance is recorded | |||||||||
---|---|---|---|---|---|---|---|---|---|
Principal inorganic constituents | Red clay (%) | Radiolarian ooze (%) | Diatom ooze (%) | Globigerina ooze (%) | Pteropod ooze (%) | ||||
(C) | (M) | (C) | (C) | (V) | (C) | (M) | (M) | ||
a Always listed as hornblende, except for one reference to glaucophane, in a blue mud, and to actinolite, in a globigerina ooze. | |||||||||
b Includes all references to monoclinic feldspar. | |||||||||
c Includes all references to triclinic feldspar. | |||||||||
d Includes three references to green mica in blue and green muds. | |||||||||
e Includes one reference to bronsite in globigerina ooze. | |||||||||
f Does not include clay minerals, iron hydroxides, or colloidal silica. | |||||||||
g May be allogenic in part. | |||||||||
1. Allogenic minerals | |||||||||
Amphibole[a] | 44 | 19 | 10 | 100 | 60 | 50 | 36 | 60 | |
Chlorite | |||||||||
Epidote | 3 | ||||||||
Feldspar, undifferentiated | 76 | 19 | 90 | 60 | 90 | 73 | 38 | 50 | |
orthoclase[b] | 40 | X | |||||||
sanidine | 10 | 18 | 10 | ||||||
plagioclase[c] | 29 | 80 | 20 | 10 | |||||
Garnet | 1 | 20 | X | ||||||
Magnetite | 89 | 23 | 100 | 80 | 29 | 40 | |||
Mica, undifferentiated | 27 | 40 | 10 | 40 | 6 | 26 | 64 | 70 | |
white mica | |||||||||
black mica[d] | 10 | 20 | |||||||
Olivine | 7 | 40 | 16 | 10 | |||||
Pyroxene, undifferentiated | X | ||||||||
enstatite | X | ||||||||
hypersthene[e] | |||||||||
augite | 61 | 13 | 30 | 40 | 12 | 70 | 12 | 40 | |
Quartz | 30 | 58 | 80 | 80 | 42 | 68 | 70 | ||
Tourmaline | 4 | 20 | X | ||||||
Zircon | 4 | 20 | X | ||||||
2. Authigenic minerals[f] | |||||||||
Analcite | 10 | ||||||||
Glauconite | 9[g] | 14[g] | 20 | 6 | 11 | 13 | |||
Manganese grains, nodules | 79 | 43 | 70 | 31 | 6 | ||||
Palagonite | 31 | 9 | 40 | 20 | 8 | 30 | 40 | ||
Phillipsite | 14 | 40 | X | ||||||
Phosphate | X | ||||||||
3. Rock fragments, etc. | |||||||||
Crystalline, sedimentary | 7 | 60 | 12 | 9 | |||||
Volcanic, glass | 64 | 23 | 70 | 80 | 70 | 60 | 65 | ||
lapilli, scoria | 16 | 10 | 20 | ||||||
pumice | 49 | 8 | 20 | 40 | 30 | 40 | 30 | ||
rock fragments | |||||||||
4. Magnetite, other (cosmic) | |||||||||
spherules | 11 | 30 | X |
The development of X-ray methods for determining the crystal structure has opened up a new field in the study of fine-grained material and the application of this technique (Revelle, 1936, Mehmel 1939) has shown that the fine clayey material is largely crystalline. Methods of identification based on X-ray spectroscopy (Mehmel, 1939) and upon certain physical and chemical tests such as the temperature-dehydration characteristics and the base-exchange capacity have made it possible to identify many of the constituents of the clay fraction. The general problems in this field have been reviewed by Grim (1939) and Kelley (1939). Revelle (1936) and Correns et al (1937) have shown the presence of quartz, calcite, and other minerals, as well as a group of substances generally known as “clay minerals” in the fine fraction of marine sediments.
Clay minerals have the following characteristic properties. Within any group there is a range in chemical composition due to isomorphous replacement. They generally contain a large amount of water partly bound as water of crystallization and partly by surface and interlattice adsorption. The platelike crystal units are mechanically weak and easily broken down and may be ruptured by the process of dispersion. Many of the clay minerals possess the property of base-exchange. In base-exchange, cations in a solution may enter the crystal structure and replace a stoichiometric equivalent of another ion which passes into the solution. For this reason it is possible to have hydrogen, sodium, potassium, calcium, and other clays, where these elements are the replaceable cations. The physical properties of such clays depend upon the replaceable cation or cations. For example, sodium clays are sticky, impermeable, and readily dispersed, whereas calcium clays are relatively granular, porous, and difficult to disperse.
The replaceable cations can be arranged in a series with decreasing replacing power:

Grim (1939) considers that there are three important groups of clay minerals as well as certain miscellaneous types that do not belong to the major groups. Under natural conditions mixtures of clay minerals usually occur.
Kaolinite Group. The chief member is kaolinite which has the formula (OH)8Al4Si4O10. Other rarer forms also belong in this group.
Montmorillonite Group. The clay mineral of this name has the formula (OH)4Al4Si8O20(xH2O). The others in this group are beidellite, nontronite, and saponite. Magnesium is usually found in montmorillonite; in beidellite the SiO2:Al2O3 molecular ratio is 3 instead of 2; in nontronite, iron replaces some of the aluminum and in saponite, magnesium replaces some of the aluminum.
Illite Group. This group has the general formula

Miscellaneous Clay Minerals. In this category are placed halloysite [(OH)8Al4Si4O10] and hydrated halloysite [(OH)16Al4Si4O6]. The noncrystalline mutual solutions of silica, alumina, and water, that may also contain some bases and for which the term allophane has been proposed, are placed in this group.
The data on the physical and chemical properties of clay minerals have been assembled by Grim (1939). The base-exchange capacity, expressed as milliequivalents per 100 g, is greatest for montmorillonite (60 to 100), intermediate for illite (20 to 40), and least for kaolinite (3 to 15). Little or nothing is known about the other types.
The clay minerals are known to result from the weathering of rocks under the action of water and its dissolved substances, particulary carbon dioxide. Plants and the products of organic decomposition also contribute to the breakdown of the rock fragments. The evidence now available indicates that the character of the residual minerals depends more upon the conditions or environment of weathering than upon the type of rock attacked.
The investigations of Mehmel on the Meteor samples and of Revelle on the Carnegie samples have shown the presence of a variety of clay minerals. The sediments of the Equatorial Atlantic contained kaolinite, montmorillonite, and hydrated halloysite, and a muscovitelike clay. Revelle obtained essentially the same results, and in addition found that certain deposits of the South Pacific contained appreciable amounts of noncrystalline siliceous material. From this evidence it would appear that at least part of the clay fractions are not formed on the sea bottom but represent the products of subaerial weathering carried into the sea
There is no sharp change in mineralogical composition or properties in passing from known terrigenous deposits to the pelagic deposits.
There is no progressive change in the mineralogical constituents with depth in cores from pelagic deposits. If submarine weathering was active, changes with time should occur which would be reflected in the cores.
The variety of fine-grained minerals indicate a number of sources, as would be found in terrigenous debris.
As stated elsewhere (p. 958), fine-grained material, particularly in dilute suspensions, is not necessarily flocculated by mixing with sea water, hence can be transported for great distances in the sea.
Although the present evidence does not preclude the formation of some clay minerals from volcanic debris on the sea bottom, there is considerable evidence which indicates that much of the clay fraction found in marine deposits, both nearshore and pelagic, is of terrigenous origin. The detection of this finely divided material in the water and the determination of its distribution in the ocean waters will be the conclusive argument in deciding upon the source of the red clay and the nonorganic fraction of the pelagic oozes.
Many of the clay minerals in the sediments possess the property of base-exchange. The question of whether or not any exchange takes place when terrigenous clays come in contact with sea water is unsettled. Kelley and Liebig (1934) found that clay minerals treated with sea water attained an equilibrium in which magnesium formed the principle replaceable base, followed by sodium, calcium, and potassium. In the sea water the order of abundance of these cations is sodium, magnesium, calcium, and potassium (p. 176). The high proportion of magnesium in the clays is due to its high replacing power, while sodium takes second place because of its abundance in the water. Revelle (1936) found that the base-exchange capacity of three clay samples was relatively high (27, 34, and 39 milliequivalents per 100 g) and that the size fractions less than 1.5 microns had even higher values. In the two cases reported these were 53 and 58 milliequivalents per 100 g. Magnesium was the most abundant replaceable cation. In the whole samples, the ions were in the order magnesium, calcium, sodium, potassium, but in the material of diameters less than 1.5 microns the sodium exceeded the calcium.
It should be pointed out that leaching with pure water or treatment with any salt solution, such as those used as dispersing agents, will tend to bring about certain changes in the exchangeable cations in the clay minerals. Hence, chemical analyses or studies of the base-exchange properties must be made on unwashed samples of the sediments.
Chemical Composition of Pelagic Sediments. As pointed out above, the composition of pelagic deposits covers a wide range and no two specimens will ever be identical in physical or chemical composition. Therefore it is desirable to turn our attention once more to the three extreme types of material commonly found in pelagic deposits, namely, calcareous remains, siliceous skeletal structures, and the fine clay material.
In table 48 (p. 231) are given examples of the chemical composition of various types of skeletal structures. Revelle (1936) analyzed foraminiferal tests carefully separated from five samples of globigerina ooze. The maximum, minimum, and average amounts of the various determined constituents are given in table 110. The average CaCO3 content is 96.2 per cent. According to Revelle the high iron content may be due to changes occurring after deposition. The composition of the siliceous skeletal material may be taken to be hydrated silica (SiO2xH2O). Hence, the organic constituents will yield two extreme types of deposits which may be either virtually all CaCO3 or all SiO2. Traces of many other elements are undoubtedly represented, and there are many minor anomalies in the composition of skeletal material. For example, among the Radiolaria there are forms that secrete strontium carbonate and others that have skeletons of complex silicates, but these will not materially affect the general picture.
Constituents | Maximum | Minimum | Average |
---|---|---|---|
* Only two determinations. | |||
SiO2 | 0.72 | 0.08 | 0.47 |
Al2O3[*] | 1.35 | 0.22 | 0.78 |
FeO,Fe2O3 | 1.68 | 0.51 | 1.08 |
MgO | 0.16 | 0.10 | 0.13 |
CaO | 54.52 | 53.12 | 53.82 |
CO2 | 43.10 | 41.69 | 42.37 |
Ignition loss[*] | 0.87 | 0.78 | 0.72 |
99.47 |
When considering the composition of the fine-grained clay material the question arises as to whether or not it is possible to consider a single type of composition or whether there are a number of varieties. The available data indicate that the first approach is a valid one. In table 111 are given three analyses of “pure” red clays. These have been adjusted to a basis as being free from salt, decomposable organic matter, calcium carbonate, and water. The analysis by Steiger (Clarke, 1924) was made on a composite sample prepared by Sir John Murray from
Constituents | Composite of 51 samples (Steiger) | Average of 10 Pacific Ocean samples (Revelle) | Atlantic Ocean sample (Correns) | Average of igneous rocks (Clarke, 1924) |
---|---|---|---|---|
SiO2 | 54.48 | 55.95 | 53.27 | 59.14 |
TiO2 | 0.98 | 0.83 | 0.98 | 1.05 |
Al2O3 | 15.94 | 18.48 | 23.74 | 15.34 |
Cr2O3 | 0.012 | 0.055 | ||
Fe2O3 | 8.66 | 8.16 | 2.65 | 3.08 |
FeO | 0.84 | 3.39 | 3.80 | |
Ni,CoO | 0.039 | 0.025 | ||
MnO | 0.31 | 0.124 | ||
MnO2 | 1.21 | 0.83 | ||
MgO | 3.31 | 2.95 | 0.38 | 3.49 |
CaO | 1.96 | 0.45 | 2.48 | 5.08 |
SrO | 0.056 | 0.022 | ||
BaO | 0.20 | 0.06 | 0.055 | |
K2O | 2.85 | 3.35 | 2.36 | 3.13 |
Na2O | 2.05 | 1.32 | 2.27 | 3.84 |
V2O3 | 0.035 | 0.026 | ||
As2O3 | 0.001 | tr. | ||
MoO3 | tr. | |||
P2O5 | 0.30 | 0.18 | 0.02 | 0.30 |
CuO | 0.24 | 0.015 | ||
PbO | 0.008 | 0.002 | ||
ZnO | 0.005 | 0.005 | ||
ZrO | 0.14 | 0.04 | ||
H2O | 7.04 | 7.30 | 8.15 | 1.15 |
Total % | 100.00 | 100.00 | 100.00 | 99.77 |
Total Fe as Fe2O3 | 9.59 | 8.16 | 6.18 | 7.31 |
Total Mn as MnO2 | 1.21 | 0.83 | 0.38 | 0.15 |