The North Atlantic Ocean
Water Masses of the North Atlantic Ocean. The character of the water masses in the North Atlantic Ocean is illustrated in the T-S diagram in fig. 183. Ten stations scattered over the North Atlantic have been selected, the locations of which are shown in the inset map. Observations from the upper 100 m have been omitted. All stations are located at such distances from the coast that local waters are not considered.
The striking features of the presentation are that in the North Atlantic Ocean one has to deal principally with two typical water masses: the North Atlantic Central Water, characterized by a nearly straight T-S curve between the points T = 8°, S = 35.10‰, and T = 19°, S = 36.70‰; and a deep and bottom water, which is characterized by temperatures between 3.5° and 2.2°, and salinities between 34.97 ‰ and 34.90 ‰. Between these two typical water masses are found other
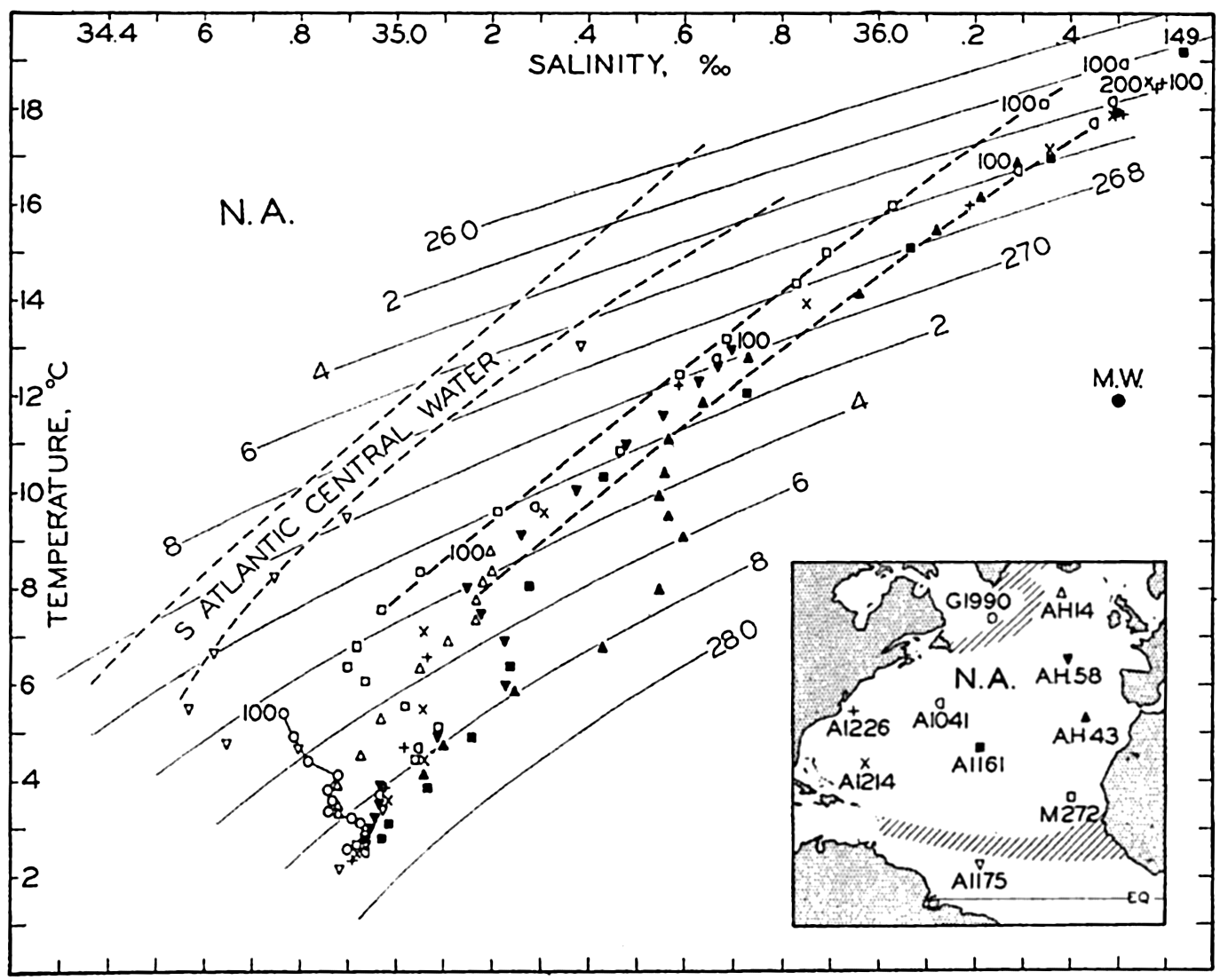
Temperature-salinity relation in the North Atlantic Ocean. The depths of the shallowest values are indicated. Large squares represent winter surface values in the northwestern Atlantic. Inset map shows location of stations. Abbreviations: A = Atlantis, AH = Armauer Hansen, G = General Greene, M = Meteor, N.A. = North Atlantic Central Region, M.W. = Mediterranean Water.
[Full Size]
The North Atlantic Central Water evidently spreads over a very large area, because it is typically present at all stations shown in the map except at station G 1990 to the south of Cape Farewell on Greenland, and at station A 1175 in latitude 6°50′N. At station AH 14a, to the south of Iceland, North Atlantic Central Water is found although no water occurs of a temperature above 9°. There is a remarkable agreement between the upper water at such widely separated stations as AH 58 to the west of the Bay of Biscay and A 1226 in the Gulf Stream region to the east of Cape Hatteras. At station A 1175 water of a different character is present, namely upper water which originates in the South Atlantic Ocean and has been carried across the Equator, but this water does not appear to exercise any great influence on the large body of North Atlantic water. The water at station G 1990 to the south of
At intermediate depths, three other types can be pointed out. At station A 1175 Antarctic Intermediate Water is present which has its origin in the South Atlantic Ocean. Upon entering the North Atlantic, this water mixes with the North Atlantic masses and appears to exercise an influence on water which has a σt of approximately 27.4. Another type of intermediate water is present at the two northern stations and is characterized by a salinity of about 34.88 ‰ and a temperature of 3.5°. This water represents the corresponding Arctic Intermediate Water which, however, is formed in small quantities only and exercises a very limited influence outside of the region where it originates.
At three of the stations in the eastern Atlantic, AH 43, AH 58, and M 272, high salinities are found at intermediate depths. These high salinities result from the spreading out of Mediterranean water which, off the Strait of Gibraltar, according to Wüst (1935), has a temperature of 11.9°, a salinity of 36.50‰, and a σt of 27.78. The Mediterranean water, as defined by these values, is indicated in the figure by the point marked MW. The form of the T-S curves clearly indicates that this water spreads in the Atlantic Ocean between the 27.6 and 27.8 σt surfaces. Here again one has to deal with a type of water which has not been formed in the open North Atlantic Ocean but in one of the adjacent seas and which shows up over large areas, as clearly demonstrated by Wüst's charts.
The deep and bottom waters are of a remarkably uniform character all over the Atlantic Ocean, as is evident from the excellent agreement between values of temperature and salinity which have been determined in different regions by different expeditions. The deep water has its origin in the most northern part of the North Atlantic Ocean, but the bottom water is probably somewhat mixed with bottom water of antarctic origin. We shall return to that question when discussing the deep-water circulation.
The large body of North Atlantic Central Water must have been formed in the North Atlantic Ocean. Iselin (1939) has shown that this water has probably attained its characteristic temperatures and salinity when in contact with the atmosphere. Making use of Böhnecke's (1936) charts of surface salinity and surface temperature of the North Atlantic Ocean in late winter, Iselin plotted temperature-salinity values from the surface in a diagram, together with typical T-S curves in mid-ocean. These surface values, which represent the characteristic T-S relation in a horizontal direction, nearly coincide with the vertical T-S curves, and by selecting other corresponding values at the surface one could obtain complete coincidence. This agreement supports the conclusion that the central water mass is formed by sinking of surface water and
The thickness of the North Atlantic Central Water is closely related to the character of the currents in the North Atlantic Ocean. Within a region of a strong current the thickness will be great on the right-hand side of the current and small on the left-hand side, according to the general rule that in the Northern Hemisphere light water is present on the right-hand side of a current.
The Currents of the North Atlantic Ocean. The system of currents in the North Atlantic (chart VII) is dominated by the North Equatorial Current to the south and the Gulf Stream system to the north. The North Equatorial Current flows from east to west in the trade-wind region and is fed by the southeasterly currents off the west coast of North Africa. Corresponding to flow from the northwest, water of relatively high density and low temperature is found off the African coast, as is evident from the charts of surface temperatures (charts II and III). The temperature close to the coast is also lowered by upwelling from moderate depths due to the action of prevailing northwesterly winds, but this upwelling does not exercise influence as widespread as does the corresponding upwelling off the coasts of southwest Africa or, particularly, as does that off the west coasts of North and South America. Details as to the upwelling off northwest Africa are still lacking, but the results of the work of the Meteor in these waters in 1937 (Defant, 1937a) can be expected to give much interesting information.
The wide North Equatorial Current runs, as already stated, from east to west, but does not follow an absolutely straight course. Schumacher (1940) points out that north of 15°N, on most of the monthly charts of the surface currents of the North Atlantic Ocean which he has prepared by means of ships’ observations, the North Equatorial Current bends to the north (to the right) when approaching the mid-Atlantic Ridge and to the south (to the left) after having passed the ridge. Schumacher also draws attention to the fact that on Defant's chart (1936b) of “average currents in the upper layers as derived from the inclination of the discontinuity layer in the troposphere,” these bends are much more conspicuous and that they appear reduced at the surface, probably owing to the superimposed pure wind drift. The maximum deflection to the north occurs directly above the mid-Atlantic Ridge and it seems therefore that the bends are due to the crossing of the ridge,
In the western part of the Atlantic Ocean the North Equatorial Current joins the branch of the South Equatorial Current which has crossed the Equator and which, according to fig. 183, p. 669, carries characteristically different water masses. Mixing takes place between these water masses and the corresponding North Atlantic water, and the waters in the Caribbean Sea are therefore intermediate in character (table 77, p. 639). Thus, the part of the North Equatorial Current which continues into the Caribbean Sea carries water which is mixed with water of South Atlantic origin, whereas the northern branch of the North Equatorial Current which flows along the northern side of the Great Antilles as the Antilles Current carries water which is identical with that of the Sargasso Sea.
The North Equatorial Current terminates in the current through the Yucatan Channel and the Antilles Current. The continuation of these currents represents the beginning of the Gulf Stream system which dominates the circulation of a great part of the North Atlantic Ocean. Following the nomenclature of Iselin (1936), the term “Gulf Stream System” is used to include the whole northward and eastward flow beginning at the Straits of Florida and including the various branches and whirls found in the eastern North Atlantic that can be traced back to the region south of the Newfoundland Banks. This system can be subdivided into the three following parts:
The Florida Current. The northward-moving water from the Straits of Florida to a point off Cape Hatteras where the current ceases to follow the continental slope. The Florida Current can be traced directly back to the Yucatan Channel, as explained on p. 642, because the greater part of the water flowing through this strait continues on the shortest route to the Straits of Florida and a small amount only sweeps into the Gulf of Mexico, later to join the Florida Current. After having passed the Straits of Florida the current is reinforced by the Antilles Current, but the name “Florida Current” is retained as far as to Cape Hatteras.
The Gulf Stream. The mid-sector of the system, from the region where the current first leaves the continental slope off Cape Hatteras to
― 673 ―the region to the east of the Grand Banks in about long. 45°W where the stream begins to fork. This application of the name “Gulf Stream” represents a restriction of the popular term, but such a restriction is necessary in order to introduce clear definitions.The North Atlantic Current. The name is used as a general term covering all the easterly and northerly currents of the North Atlantic from the region to the east of the Grand Banks where the Gulf Stream divides. The branches of the North Atlantic Current are often masked by shallow and variable wind-drift surface movements, which have become commonly known as the North Atlantic Drift.
The terminal branches of the Gulf Stream System are not all well known, but among the major ones are the Irminger Current, which flows towards the west to the south of Iceland, and the Norwegian Current, which enters the Norwegian Sea across the Wyville Thomson Ridge and can be traced ultimately into the Polar Sea (p. 661). Other more irregular branches which turn to the south have been examined by Helland-Hansen and Nansen (1926), who find that they terminate in great whirls off the European coast.
The Florida Current. The energy of the Florida Current appears to be derived directly from the difference in sea level between the Gulf of Mexico and the adjacent Atlantic coast, the observed difference between Cedar Keys and St. Augustine being 19 cm. (p. 642). Assuming that this hydrostatic head accounts for all of the energy, and assuming frictionless flow, Montgomery (1938) finds that the velocity through the Straits of Florida should be 193 cm/sec, which is somewhat higher than the average velocity at the center of the current. The difference in level is probably maintained by the trade winds, and the energy of the Florida Current is therefore derived from the circulation of the atmosphere.
Within the current flowing through the Straits of Florida, the distribution of density must adjust itself in the usual manner; that is, the lighter water must be found on the right-hand side of the current and the denser water on the left-hand side, and the sea surface, instead of coinciding with a level surface, must accordingly rise towards the right-hand side of the current. In the case of the Florida Current this rise amounts to about 45 cm, sea level at the coast of Cuba being about 45 cm higher than at the American mainland (Dietrich, 1936).
The character of the currents in the Straits of Florida was established by the outstanding measurements made in the years 1885 to 1889, by the United States Coast and Geodetic Survey from the survey vessel Blake, commanded by J. E. Pillsbury. Pillsbury's observations of currents, carried out from a vessel anchored in deep water in a swift stream, are among the classical data in physical oceanography, not so much because they give complete information as to the average currents, but mainly because they made possible a convincing demonstration of the
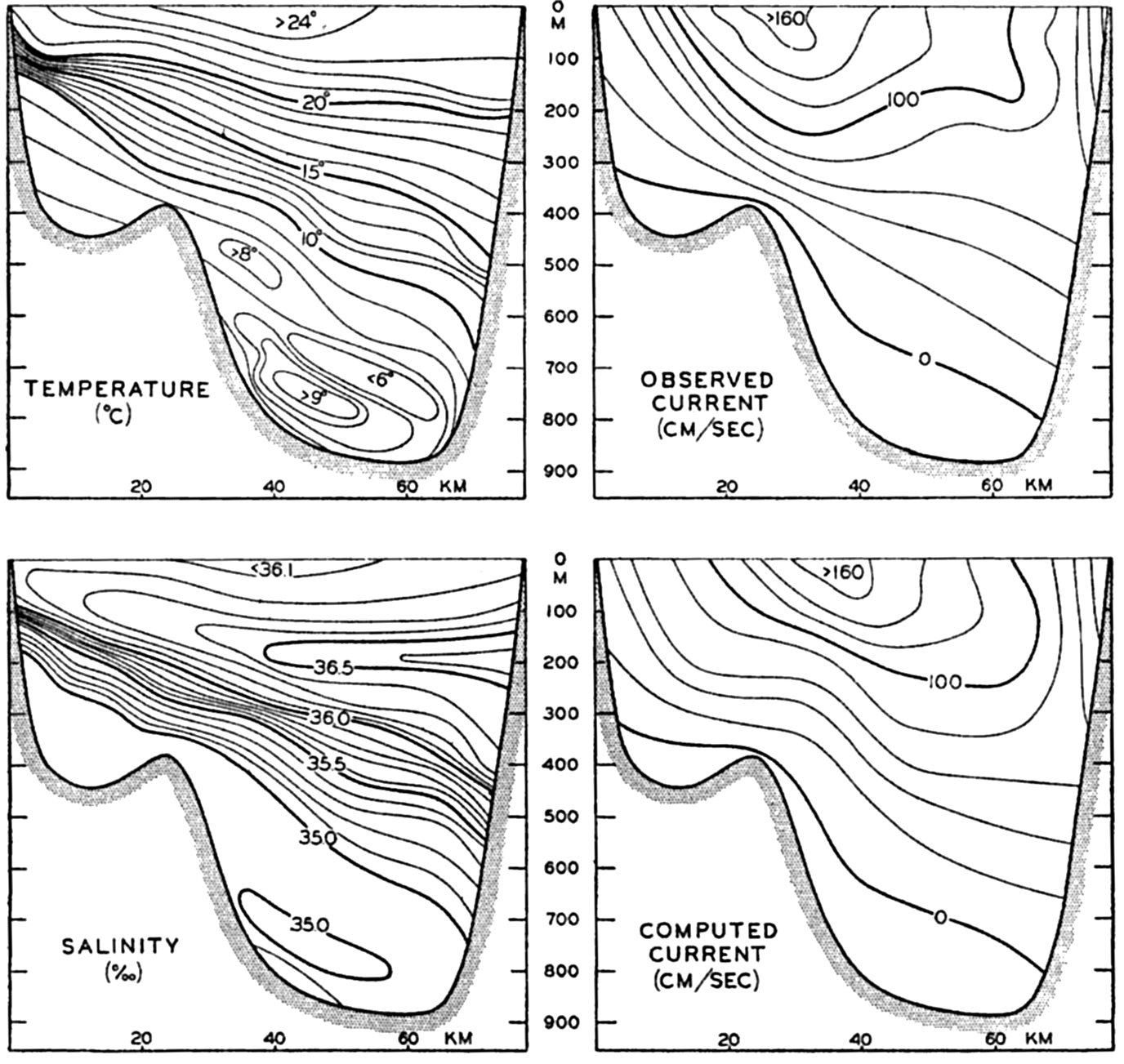
Left: Observed temperatures and salinities in the Straits of Florida. Right: Velocities of the current through the Straits according to direct measurements and according to computations based on the distributions of temperature and salinity (after Wüst).
[Full Size]
On the basis of measurements and computations Wüst finds that the average transport of water through the Straits of Florida is 26 million m3/sec. The transport probably shows an annual variation and may differ from year to year, but so far little is known about such fluctuations (Montgomery, 1938, 1941, Iselin, 1940).
In its further course the Florida Current closely follows the continental slope, flowing most swiftly directly along the slope. The shallow coastal waters to the left of the Florida Current remain more or less at rest; often the transition from these waters to the blue waters of the Florida Current is so abrupt that the border of the Florida Current can be seen as a line stretching from horizon to horizon. After emerging from the Straits of Florida, the current is soon joined by the Antilles Current which, according to Wüst (1924), carries about 12 million m3/sec. Owing to the moderate depth to the bottom, the current remains relatively shallow, not more than about 800 m deep, and carries no water colder than 6.5° until it leaves the Blake Plateau in about lat. 33°N. According to Iselin (1936) the current increases steadily in volume by absorption of Sargasso Sea water, and as it leaves the Blake Plateau both the depth and the volume suddenly increase as a result of the joining in of water of a temperature considerably below 8° which comes from the southwestern Sargasso Sea.
The Gulf Stream. The middle portion of the Gulf Stream System, for which the name Gulf Stream is retained, continues as a well-defined and relatively narrow current which, in contrast to the Florida Current, flows at some distance beyond the continental shelf. To the right of the current is the Sargasso Sea water, as previously, but to the left are now found two water masses, the coastal water which covers the shallow shelf areas and the slope water which, at temperatures between 4° and 10°, is very similar to the Gulf Stream water (Iselin, 1936) but at higher temperatures is of lower salinity. Within the upper layers of the slope water great seasonal variations in temperature and salinity occur and, in addition, eddies of Gulf Stream water occasionally intrude.
The surface velocities of the Gulf Stream are very high, the computed values reaching, in lat. 36°N, long. 73°W, more than 120 cm/sec (Iselin, 1936) and in lat. 38°N, long. 69°W, 140 cm/sec (Seiwell, 1939). On the
The dynamics of the Florida Current and the Gulf Stream, particularly the downstream increase in volume as far as Cape Hatteras, is not clearly understood. Rossby (1936) has compared the Florida Current and its continuation, the Gulf Stream, to a wake stream which emerges from the Straits of Florida, and has examined the effect on such a stream of stresses due to lateral mixing. In a wake stream in homogeneous water the momentum transport remains constant whereas the volume (mass) transport increases downstream, the increase being due to inflow from the sides. Expanding the theory to a stratified medium, Rossby finds that a “compensation current” in the direction of flow must develop on the right-hand side of the wake stream, whereas to the left a counter current in the opposite direction must appear, and this picture agrees in general with the pattern of the Gulf Stream and its surroundings. Another important aspect of the theory is that owing to the lateral stresses a transverse circulation should develop, water being absorbed from the oceanic areas to the right of the current and discharged into the countercurrent to the left. Such a mechanism would account for the presence of eddies of Gulf Stream water in the slope current, but Defant (1937b) and Ekman (1939) have warned against immediate acceptance of the theory because several of the necessary assumptions appear not to be fulfilled. Regardless of whether the theory is confirmed or disproven, it has been greatly stimulating, particularly because of its emphasis on the importance of lateral mixing. In this connection the possibility may be mentioned that the developments of the counter current and the transverse circulation may not necessarily be associated
Locality | Sea level (cm) | Mean | Distance along coast (km) | Slope | |
---|---|---|---|---|---|
Avers, 1927 | Rappleye, 1932 | ||||
St. Augustine, Fla. | 0 | 0 | 0 | 0 | |
Fernandina, Fla. | |||||
Brunswick, Ga. | |||||
6 × 10−8 | |||||
Norfolk, Va | 4 | 7 | 6 | 1000 | |
35 × 10−8 | |||||
Cape May, N.J. | 16 | 24 | 20 | 1400 | |
Atlantic City, N.J. | |||||
Fort Hamilton, N.J. | |||||
13 × 10−8 | |||||
Boston, Mass. | 25 | 30 | 28 | 2000 | |
Portland, Me. | |||||
12 × 10−88 | |||||
Halifax, Nova Scotia | — | 35 | 35 | 2600 |
A satisfactory theory of the Gulf Stream must not only account for the increase in volume transport in the direction of flow and the fact that this increase takes place without evidence of strong inflow from the southeast, but it also must account for another important feature which has been given considerable attention (Dietrich, 1937b) without having been explained satisfactorily (Ekman, 1939). Precise leveling along the American east coast shows that the mean sea level increases towards the north from St. Augustine, Florida, to Halifax, Nova Scotia, the most conspicuous increase taking place directly north of Cape Hatteras. Table 81 summarizes the results of the precise leveling, according to Avers (1927) and Rappleye (1932). In the table the sea level along the coast has been referred to that on the coast of Florida and southern Georgia, values from stations less than 200 km apart having been eombined as averages. The distances along the coast from Florida-Georgia are entered and also the values of the slope of the sea surface. These values are of the same order of magnitude as those derived from
If this upward slope were due to the distribution of mass in the ocean, the average density of the Gulf Stream water off the continental shelf would have to decrease in the direction of flow and the Gulf Stream would have to flow uphill. Dietrich (1937b), however, has shown that along the continental slope the distribution of density does not indicate any rise of the sea surface if the topography of the surface is referred to the oxygen minimum layer, and the same is true if the topography of the sea surface is referred to the 2000-decibar surface. These conclusions are not altered by taking into consideration the small effect of differences in atmospheric pressure. A discrepancy therefore exists between the results of precise leveling and the results of what Dietrich calls oceanographic leveling. It is not surprising that such discrepancies appear because, as explained on p. 407, oceanographic observations can give information only as to the topography of the sea surface relative to some selected surface in the ocean and information as to the absolute topography of the sea surface must be derived from precise leveling along the coasts.
The question now arises whether it is possible to reconcile the different observations and at the same time arrive at transport values which are compatible with the distribution of density. The results of precise leveling are so accurate that the upward slope of the sea surface north of Cape Hatteras must be taken as established. It is also established that this slope of the sea surface is not compensated for by the distribution of mass, as is the case in the Caribbean Sea, for which reason it follows that the water must actually be piled up against the coast. The lack of compensation is understood if one considers that the piling up takes place in the shallow waters along the coast and does not extend to any distance beyond the continental slope. However, a transition must exist from the region of uncompensated piling up of mass to the region where compensation can take place, and it appears reasonable to assume that the transition takes place along the edge of the continental shelf. There the sea level sinks to the value indicated by the oceanographic data and, consequently, a current to the south runs along the continental slope, following approximately the absolute contour lines of the surface. A current to the south must also flow over the shallow portion of the shelf where it flows downhill and where the balance of forces is maintained by the effect of friction.
The transports can be calculated by considering that, owing to the piling up of water along the coast, the actual profiles of the isobaric surfaces slope downwards from the coast towards the outer part of the slope water and then rise because of the distribution of density. If this consideration is correct, the computed transport of Gulf Stream water must come out too high if based on the oceanographic data alone because
This reasoning can be illustrated by certain computations based on the Atlantis section of April 17–23, 1932, using the stations 1231–1225 which have been used extensively by Iselin and Dietrich. It is assumed here that over the shelf the piling up of water leads to the sea level on the coast being 10 cm higher, that the same piling up effect is present at the border of the continental shelf, and that no effect is found beyond a distance of 40 km from the border. Furthermore, it is assumed that beyond that distance the 2000-m level is a level of no motion. On the basis of such assumptions one arrives at the following figures:
Transport to the southwest of slope water inside of station 1229 | 19 million m3/sec. |
Transport to the northeast of slope and Gulf Stream water outside of station 1229 | 74 million m3/sec. |
Net transport to the northeast | 55 million m3/sec. |
According to this computation the net transport of the Gulf Stream is of 55 against 74 million m3/sec if the southward motion of the slope water is disregarded. A net transport of about 55 million m3/sec is in agreement with the previous conclusion (p. 676) that not more than 15 to 20 million m3/sec circulate in the gyral to the right of the Gulf Stream; and if the reasoning is correct, another gyral is present on the left-hand side within which approximately 20 million m3/sec circulate. The slope water on the coastal side of the Gulf Stream is essentially of the same character as the Gulf Stream water, but has a slightly lower salinity owing to admixture with coastal water. The southward motion directly off the continental shelf is not associated with high surface velocities because one has to deal with a “slope current,” and on the assumptions made the velocity of the current is about 10 cm/sec.
Figure 185 gives a schematic picture of the conditions which have been described. The lines provided with arrows represent transport lines, each line corresponding to a transport of about 10 million m3/sec. The two gyrals on both sides of the Gulf Stream are shown and, in addition, an eddy is indicated between the slope-water gyral and the Gulf Stream where the latter is at a greater distance from the coast. Such an eddy, rotating clockwise, has repeatedly been observed to the south of Nova Scotia (Iselin, 1936), but the clockwise rotation has been difficult to explain because the eddy was considered an offshoot of the Gulf Stream. The direction of rotation is better understood, on the other hand, if the eddy is considered as a part of the slope-water gyral. The inset diagram shows a profile of the sea surface along the line A-B. The line marked 1 represents the profile which is obtained from oceanographic observations and the line marked 2 is the profile which is assumed to exist on the basis of these observations and the results of precise leveling.
It remains to account for the piling up of the water masses on the continental shelf. It can be suggested that this piling up is maintained by the prevailing winds over a large area of the North Atlantic Ocean. Southwesterly winds over the northern parts of the North Atlantic may maintain a higher sea level along the northern borders of the ocean and, consequently, a slope of the sea surface along the eastern and western borders.
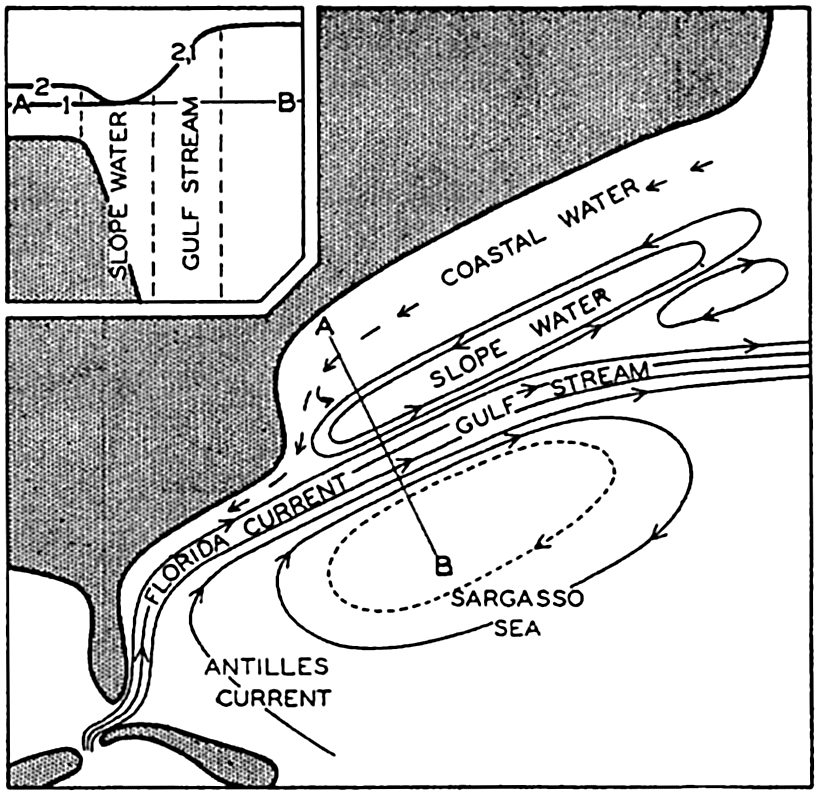
Schematic representation of the character of the Gulf Stream, taking results of precise leveling into account. Inset: Profiles of the sea surface along the line A-B. Profile 1 derived from oceanographic data only; Profile 2, from these data and the results of precise leveling.
[Full Size]
The North Atlantic Current. The North Atlantic Current represents the continuation of the Gulf Stream after it leaves the region to the east of the “tail” of the Grand Banks. Beyond this region the Gulf Stream loses its characteristics as a well-defined current and divides into branches that are often separated by countercurrents or eddies. Some of the branches turn south but others continue towards the east across the mid-Atlantic Ridge, being flanked on the northern side by waters of the Labrador Current that have been mixed with Gulf Stream water.
The contrast between the Gulf Stream and the North Atlantic Current to the north of the Azores is illustrated in fig. 186, which shows two temperature profiles on the same scale. To the left in the figure are shown the isotherms in a vertical section from Chesapeake Bay towards Bermuda according to observations at the Atlantis stations 1231–1226. The Gulf Stream is here concentrated within the narrow band in which the isotherms slope steeply downward toward the right. The section to the right in the figure runs north-northwest from the Azores to lat. 48°0N and is based on the observations on board the Altair during the International Gulf Stream Expedition in 1938 (Defant and Helland-Hansen, 1939). In this section the isotherms generally slope downward toward the south, indicating a flow towards the east, but the slope is not uniform and countercurrents or eddies are present between the east-flowing branches of the current. In the section is shown the location of the Altair cone, discovered during the expedition, which probably
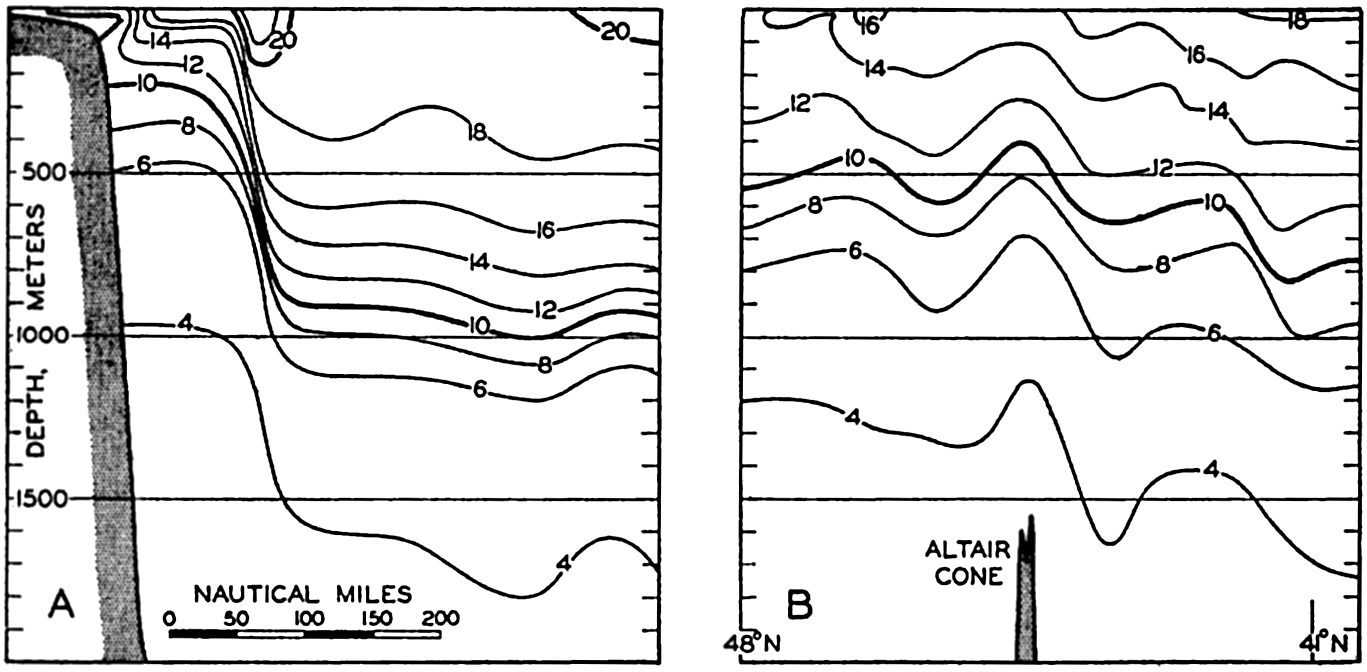
Temperature profiles across the Gulf Stream off Chesapeake Bay and across the North Atlantic Current to the north of the Azores.
[Full Size]
The intensive work conducted by the International Gulf Stream Expedition of 1938 (Defant and Helland-Hansen, 1939) clearly shows the complicated details of the oceanographic conditions. Between June 1 and 22, 1938, the German vessel Altair and the Norwegian vessel Armauer Hansen occupied 159 stations in an area of less than 100,000 square miles, and at one station the Altair anchored and made hourly observations of temperature, salinity, and currents at a number of depths between the surface and 800 m for a period of 90 hours. The dense network of stations showed even greater irregularities than one might expect. At 600 m, for example, the temperature varied between approximately 7° and 13°, and differences up to 5° were observed at distances less than 40 miles. Some of the observed features may be due to the influence of the bottom topography and others may be related to traveling disturbances. Regardless of how the features are interpreted, they do show that caution has to be exercised when drawing conclusions from a few scattered observations
A series of stations which were occupied in 1931 by the Atlantis along the mid-Atlantic Ridge in long. 30°W demonstrates that in spite of irregularities one can distinguish between two major branches of the North Atlantic Current. The northern branch flows between lat. 50° and 52°N, at the boundary between the water of the Gulf Stream System and the Subarctic Water, and carries water which represents Gulf Stream water mixed with waters of the Labrador Current. The other branch flows approximately in lat. 45°N and carries undiluted Gulf Stream water. The northern branch continues mainly towards the east-northeast and divides up. Part of the water flows across the Wyville Thomson Ridge into the Norwegian Sea (p. 653) and part turns toward the north and northwest as the Irminger Current that flows along the southern coast of Iceland. A small portion of the water of the Irminger Current bends around the west coast of Iceland (fig. 178, p. 652), but the greater amount turns south and becomes more or less mixed with the waters of the East Greenland Current. The detailed work of the Meteor in the area between Iceland and southeastern Greenland (Defant, 1936c) indicates that many eddies within which this mixing takes place remain in approximately the same locality from one year to another, and the position of the eddies may therefore be related to the configuration of the coast. The last traces of the Gulf Stream water continue around Cape Farewell where, at some distance from the coast, water of salinity above 35.00 ‰ is encountered (fig. 181, p. 663).
In the central part of the Irminger Sea, off southern Greenland, mixing between the North Atlantic water and the Labrador Sea water leads to the formation of Subarctic Water of uniform salinity close to 34.95 ‰ and a temperature which from a depth of a few hundred meters and downwards is nearly 3°C. When the surface layers are cooled in winter to temperatures below 3°, vertical convection currents develop, reaching from the surface to the bottom and leading to renewal of the deep water. Table 82 contains data from two Meteor stations which were occupied in early spring and at which nearly uniform water was present. The oxygen observations have been included in order to show the high oxygen content of the deep water. The intense mixing that takes place in winter in this area and in the Labrador Sea (p. 664) must have important bearing on the productivity of the subarctic waters.
The branch of the North Atlantic Current that crosses the mid-Atlantic Ridge in approximately lat. 45°N turns to the right and continues as an irregular flow toward the south between the Azores and Spain, sending whirls into the Bay of Biscay. No distinct currents exist in this region, as is evident from the discussions of the conditions in the eastern North Atlantic by Helland-Hansen and Nansen (1926), but a diffuse
Depth (m) | Meteor 121, March 9, 1935 Lat. 56°037′N, Long. 44°054.5′W | Meteor 79, March 30, 1933 Lat. 59°038′N, Long. 40°042.5′W | ||||||||
---|---|---|---|---|---|---|---|---|---|---|
Temp. (0°C) | S (‰) | σt | O2 (ml/L) | O2 (%) | Temp. (0°C) | S (‰) | σt | O2 (ml/L) | O2 (%) | |
0 | 2.82 | 34.87 | 27.80 | 7.24 | 96 | 4.07 | 34.96 | 27.76 | 6.92 | 95 |
50 | 3.01 | 34.90 | 27.81 | 7.26 | 97 | 4.07 | 34.97 | 27.77 | 6.99 | 96 |
100 | 3.09 | 34.92 | 27.82 | 7.24 | 97 | 4.06 | 34.96 | 27.77 | 6.81 | 94 |
200 | 3.17 | 34.93 | 27.82 | 6.70 | 90 | 3.98 | 34.97 | 27.77 | 6.84 | 94 |
800 | 3.26 | 34.96 | 27.83 | 6.98 | 94 | 3.76 | 34.95 | 27.77 | 6.60 | 90 |
1000 | 3.20 | 34.95 | 27.83 | 6.96 | 93 | 3.33 | 34.89 | 27.77 | 6.64 | 89 |
1500 | 3.17 | 34.93 | 27.82 | 6.99 | 94 | 3.28 | 34.94 | 27.82 | 6.39 | 86 |
2000 | 3.23 | 34.93 | 27.82 | — | — | 2.84 | 34.96 | 27.88 | 6.37 | 87 |
Transport. On the basis of the above discussion and numerous computations of transports, fig. 187 has been prepared, giving a schematic picture of the volume transport of the currents which carry North Atlantic Central Water or Subarctic Water. No lines of equal transport are entered but the different branches of the current system are indicated and the approximate volume transport in millions of cubic meters per second is stated. The presentation has been derived by computing the transport of water of temperature higher than 7° between a number of selected stations north of lat. 20°N, and adjusting the figures in order to take the continuity of the system into account. To the northwest of a line which can be drawn roughly between the Straits of Florida and the English Channel it has been assumed that the 2000–decibar surface could be taken as a surface of no motion, but to the southeast of that line it has been assumed in general that the 7° isothermal surface was a surface of no motion. This treatment was necessary because the condition of continuity has to be satisfied and because a reversal of the direction of flow appears to take place below the 7° isothermal surface over large parts of the southeastern area. It is not claimed that the picture in fig. 187 is accurate in details, but it is believed that it gives an approximately correct
The selected surface of no motion has several features in common with the corresponding surface which Defant (1941) arrived at by using the third method discussed on p. 457, without taking the equation of continuity into account. Defant's results as to the prevailing currents differ somewhat, however, from ours, particularly along the north coasts of the Antilles where he finds currents toward the southeast dominating, whereas according to our analysis the currents are directed toward the northwest in agreement with other generally accepted results.
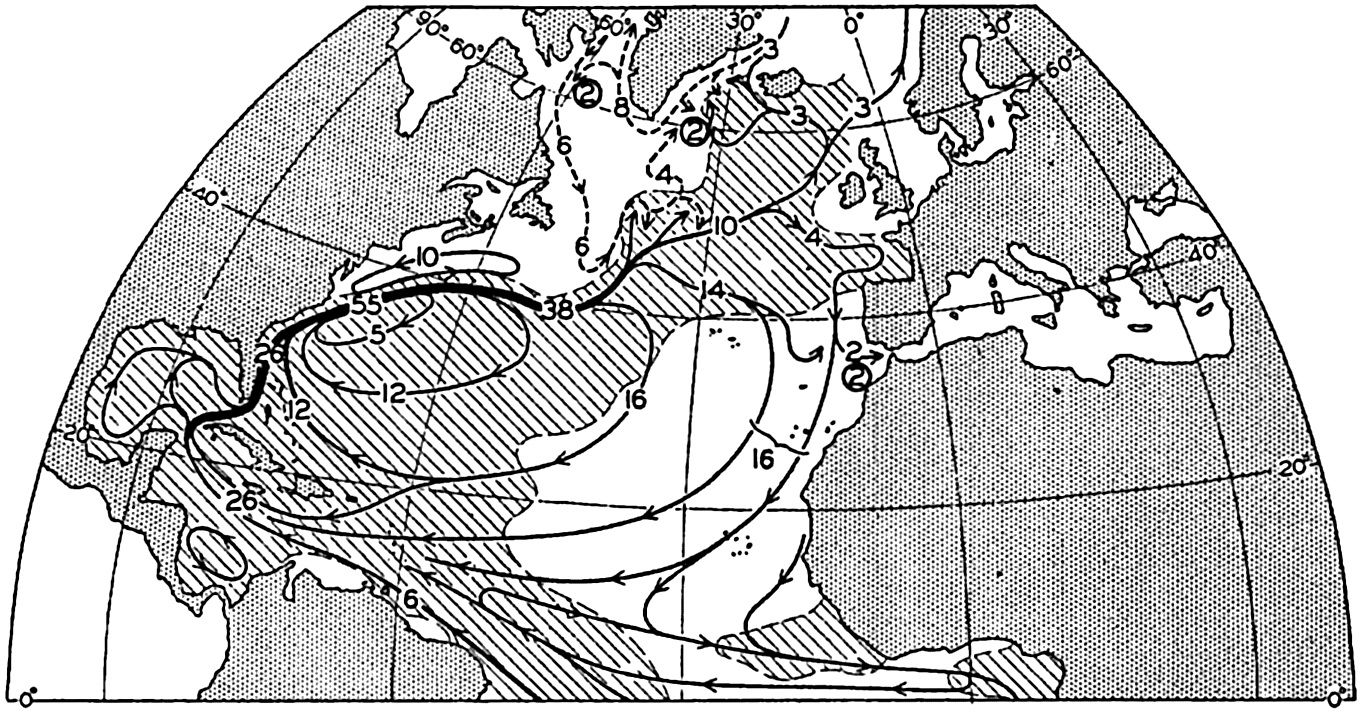
Transport of Central Water and Subarctic Water in the Atlantic Ocean. The lines with arrows indicate the direction of the transport, and the inserted numbers indicate the transported volumes in millions of cubic meters per second. Full-drawn lines show warm currents, dashed lines show cold currents. Areas of positive temperature anomaly are shaded.
[Full Size]
The representation in fig. 187 shows the Florida Current and the Gulf Stream as the only well-defined currents of the Atlantic Ocean. It also shows that the greater amount of the waters of the Gulf Stream turns south before reaching the Azores and circulates around the Sargasso Sea.
In the figure the areas are shaded in which the average surface temperature is higher than the general average for that latitude. The shaded portions therefore represent the areas with positive temperature anomalies as referred to the average temperatures of the Atlantic Ocean, and the unshaded portions represent the areas of negative temperature anomalies. As should be expected, water which is transported from lower to higher latitudes is relatively warm, whereas water which is transported from higher to lower latitudes is relatively cold. If the velocity distribution within the different branches of the currents were known it would be possible to compute the net amounts of heat which are carried
Another characteristic of the system of currents is brought out by a comparison between the transports and the temperatures off Chesapeake Bay and to the north of the Azores (fig. 186). The Gulf Stream off Chesapeake Bay transports large volumes of water of temperatures above 16° but the North Atlantic Current to the north of the Azores carries only small amounts of water as warm as 16°. This apparent reduction in temperature must be due to the fact that the warmer waters of the upper layers have been carried south before reaching the Azores, whereas the somewhat colder waters at greater depths have continued toward the east. If such is the case, the law of the parallel solenoids is not fulfilled, owing possibly to cooling in the direction of flow (Parr, 1936).
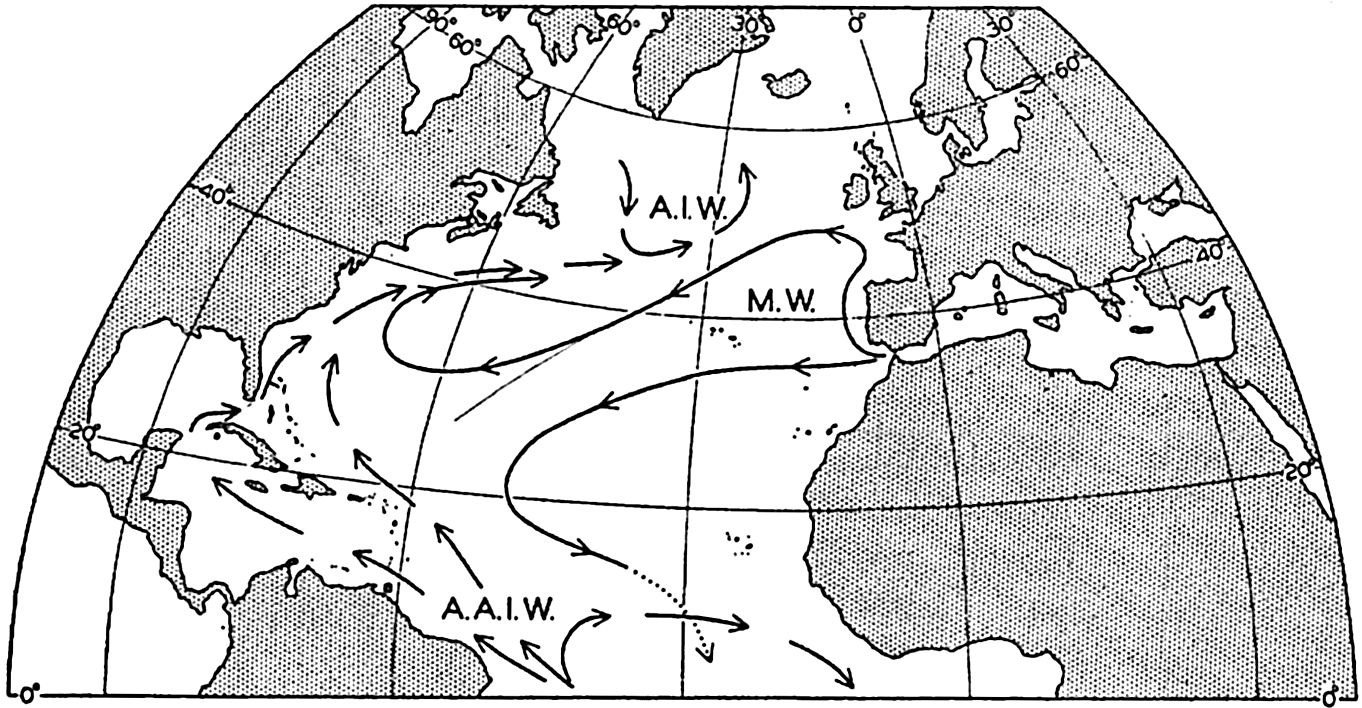
Approximate directions of flow of the intermediate water masses of the North Atlantic. A.I.W., Arctic Intermediate Water; M.W., Mediterranean Water; A.A.I.W., Antarctic Intermediate Water.
[Full Size]
As previously mentioned (p. 629), 6 million m3/sec of South Atlantic Upper Water enter the North Atlantic Ocean along the coast of South America. Correspondingly, 6 million m3/sec of North Atlantic water sink in different localities and return to the South Atlantic as a deep-water flow. The three regions where sinking takes place and the amounts of water sinking from the surface are shown by circles and inserted numbers that represent rounded-off values. About 2 million m3/sec sink outside the Strait of Gibraltar and about the same amount sinks in the Labrador Sea. Both of these values are based on fairly accurate data (pp. 647 and 666), wherefore it follows that a similar amount sinks in the third region within which bottom and deep water is being renewed, namely the region to the southeast of southern Greenland.
The direction of flow of the different types of intermediate waters of lower temperature does not always coincide with the direction of flow of the upper water. On the basis of the character of the water and the results of dynamic calculations, fig. 188 has been prepared, giving a tentative picture of the spreading of the Arctic Intermediate Water, the Mediterranean and the Antarctic Intermediate Water. The Arctic Intermediate Water is present in the northern region only. The Mediterranean water partly bends north before turning west and partly spreads directly toward the west. Some of this water turns south and continues across the Equator below the Antarctic Intermediate Water, as indicated by the crossing of the lines of flow. The Antarctic Intermediate Water enters the North Atlantic Ocean along the coast of South America. One branch bends toward the east and south, returning across the Equator, and two other branches continue, one into the Caribbean Sea and the other along the north side of the Antilles. Mixing takes place between the different types of water and the actual pattern of flow or spreading out is therefore far more complicated than is shown. For details it is necessary to refer to the discussions by Wüst (1935) and Iselin (1936).
Oxygen Distribution. The general character of the distribution of oxygen in the North Atlantic is evident from fig. 43, p. 210 and fig. 210, p. 748. The upper layers are rich in oxygen, but at depths between 500 and 900 m an oxygen minimum layer is present except in the northern parts of the ocean. In the minimum layer the lowest values are found in lat. 15°S to 15°N, where on the eastern side of the ocean (shown in fig. 43) values below 1.0 ml/L are observed as compared to minimum values of about 3.0 ml/L on the western side (fig. 210). To the north of 30°N the contrast between the two sides of the ocean is reversed and the lowest values are found on the western side, although they do not drop much below 4.0 ml/L.
Below the oxygen-minimum layer the oxygen content increases rapidly with depth; the deep water of the North Atlantic contains very large amounts of oxygen which decrease somewhat, however, from north to south. In the region where the North Atlantic Deep Water is formed, the oxygen content is at all depths higher than 6.5 ml/L (see table 82, p. 683) and from that area the oxygen content gradually decreases to 5.5 ml/L at the Equator and less than 5 ml/L in 45°S. In the same distance the percentage saturation decreases from about 85 to 75 or 70. The southern part of the Weddell Sea is the only other oceanic area in which water at depths below 2000 m contains as much oxygen as does the deep water of the North Atlantic Ocean.