XVI. Phytoplankton in Relation to Physical-Chemical Properties of the Environment [PDF]
With the phytoplankton are included all of the passively floating plants of the sea (fig. 213). In our review of the systematic position, general structure, and methods of reproduction of the plant population comprising the floating flora of the sea, we noted that the population is composed of an assortment of organisms which, largely for convenience, were grouped under the yellow-green algae. It must be emphasized, however, that the chief components of the phytoplankton are firstly, the diatoms, and secondly, the dinoflagellates. The other autotrophic organisms are of varying importance and consist of coccolithophores, Halosphaera, silicoflagellates, and a few others. The lack of diversity of major groups in the phytoplankton as contrasted with the zooplankton is striking, but this is characteristic of the whole marine flora when compared with the marine fauna. There is no dearth of variety, however, within the restricted group represented by the diatoms and dinoflagellates. Structural variations give rise to large numbers of genera and species, but the number of species occurring in concentrations sufficient to be recorded in nontaxonomic biological surveys of the plankton in any one area is not large; and of these species, fewer yet assume numerical importance. In Puget Sound such a survey (Phifer, 1933) showed 70 species in 24 genera. Among these, 5 species in 5 genera were tychopelagic, having been swept up by currents from their normal habitat on the bottom in the littoral zone. In the Gulf of Maine and the Bay of Fundy, Gran and Braarud (1935) report 78 species of diatoms in 30 genera, and of this number 6 species in 6 genera were tychopelagic. In this region there were also 57 species of dinoflagellates in 11 genera. In warmer seas the number of dinoflagellate species is usually greater. Peters (1932) found that of 55 species of Ceratium occurring in the South Atlantic, 33 were confined to warm water. The phytoplankton may, like the zooplankton, be divided broadly into neritic and oceanic populations.
Among the neritic diatoms there are a great number which form resting spores, and this is in keeping with the greater fluctuations in salinity, temperature, and other environmental details, characteristic of
Oceanic phytoplankton may at times be abundant in the open sea, and densities of 220,000 diatoms per liter have been recorded for the open North Pacific (Allen, 1936), although concentrations are usually much less. The presence of considerable populations of both pelagic and benthic animals in deep-sea and oceanic areas gives ample evidence of offshore production, for it is only the oceanic plants, together with a few others drifting from coastal waters, that constitute the actual source of food for the offshore animals. Lohmann believed that the coastal waters, on the whole, are fifty times more productive than open ocean waters
The vital importance of the phytoplankton organisms as producers of the primary food supply of the sea has repeatedly been emphasized. Let us now examine a little closer some of the adjustments or adaptations required by the floating mode of existence and the factors influencing and controlling multiplication and growth.
METHODS OF FLOTATION
It is clear that if plant life is to exist at all in the offshore waters of more than very moderate depths, it must in some manner be maintained in the upper layers where it can float freely suspended near the surface, for only there can sunlight penetrate sufficiently to meet the requirements of photosynthesis. Once a diatom or any other autotrophic organism requiring light has sunk below this zone, it is doomed to disintegration in the lower layers unless there be prevailing in the area some sort of vertical circulation which will bring it quickly back to the surface. This may occur in some few instances, but there is a strong tendency for water to remain stratified with the lighter layers on top until strong forces overcome this stability (p. 497).
Living protoplasm is heavier than pure water, its specific weight ranging from 1.02 to 1.06, and the shells are even heavier; hence, adaptations are required to prevent or retard sinking. The rate of sinking of a body heavier than water depends upon the ratio of surplus weight to friction. Friction in turn is determined mainly by the surface area. The simplest way to obtain a relatively large surface area is to reduce the absolute size. However, adaptations have gone far beyond this simple limitation to minute size. Special structures, shapes, or other means presently to be considered have evolved to enable the small plants and animals to remain in suspension and thus to populate the vast pelagial expanses of the sea that would otherwise be sterile. Doubtless, the most important adaptation to a pelagic existence in plants is a reduction of absolute size in order to increase the ratio of surface area to volume. In plants the advantage is twofold, for it facilitates not only flotation but also absorption of plant nutrients, which in the sea are in very dilute solutions at best. As a further response to the requirements of this passive floating existence, the plants in most instances also possess structures considered to be special adaptations which aid them in keeping in suspension sufficiently long to grow and reproduce. The characteristic morphological adaptations represent forms which are diametrically opposite to streamline.
Diatoms. Let us first consider the pelagic diatoms, since they are wholly unable by voluntary means to adjust themselves with respect to depth.
The structural adaptations may be divided into four classes (Gran, 1912), and are illustrated in fig. 70, p. 296, depicting diatom types.
Bladder type, in which the cell is relatively large and the cell wall and protoplasm form a thin layer inside the shell or test, and the remainder of the cell is filled with a light cell fluid or sap. Examples are found in species of Coscinodiscus. The shape may approach that of a disc as in Planktoniella, which sinks in a zigzag course, covering a much greater distance than if it were to follow a direct line from the surface to the bottom.
The needle or hair type is long and slender and is typified by Rhizosolenia and Thalassiothrix, which sink slowly when suspended with the long axis horizontal to the pull of gravity but with increased rate when in a vertical position. The cells also may be curved or provided with ends that are beveled in such a manner that they are soon brought back into the horizontal position, once they have been displaced. In this way the sinking is accomplished in great wide circles. The same applies to Nitzschia serriata when in chains with overlapping ends.
The ribbon type is illustrated by Fragillaria and Climacodium. Here the cells are broad and flat and are attached to each other in chains in which the individual cells lie side by side in a single layer. They are not numerous in the sea.
The branched type is illustrated especially by the genus Chaetoceros, and by Corethron. Here a great many spines are grown as projections to resist sinking, and the cells are often in chains and are frequently spiraled for greater effect. They are extremely abundant in the sea.
All of the pelagic species are thin-shelled as compared with the bottom or littoral forms, and some may secrete a coating of light mucus to aid in tiding over unfavorable conditions. There are also summer and winter forms in some species. The summer forms usually have lighter shells, which is in keeping with the reduced viscosity of warmer water.
The specific gravity of diatoms may also be lessened by the presence of oil in the cells. Oil has been noted to accumulate in diatoms late in the vegetative period and, in addition to providing a food reserve, may also result in keeping them afloat while waiting return of conditions favorable for multiplication.
Many diatom species, though conforming in cell structure to either of the above types, also unite the separate cells into chains of various types. This is accomplished by siliceous cements or by mucous pads or threads (fig. 70).
Dinoflagellates. Unlike diatoms, the dinoflagellates are not strictly passive, for they are provided with two minute whiplike flagella which by almost constant undulating motion provide a means of feeble
In some dinoflagellates, for example, Triposolenia spp. and Amphisolenia spp. (fig. 74), an asymmetry is developed, resulting in a deflection of the ends of the antapical horns. During periods of rest or weak swimming, these deflected horns tend to orient the sinking body so that the long axis lies horizontal and thus provides the maximum surface and resistance against passive sinking. In Triposolenia, a descent of about ten times its body length is sufficient to swerve the organism slowly into this advantageous passive position. Here it remains at least momentarily and the frequent alternation of positions leads to a slow wavering descent.
In referring to the asymmetry discussed above, Kofoid (1906) remarks,
Had it appeared as one of the characters of a single species, or even of several, it would be dismissed as one of those usual chance vagaries of structure which so often crop out in the diagnostic features of a species. Its occurrence in the two genera named and suggestions of an analogous structural feature in some other genera of dinoflagellates is indicative of a more profound relationship to the welfare of the organisms in which it appears.
FACTORS OF PHYTOPLANKTON PRODUCTION: I
In the study of plankton, the production is generally understood as being the amount of organic matter produced by the phytoplankton under a unit area of sea surface or in a unit volume of water during a given
The principal factors controlling productivity have been reviewed by Braarud (1935) and we follow his scheme with some modifications in outlining the analysis of the direct and indirect factors. Under the heading of direct factors are included (a) direct primary factors that operate directly on the growth and proliferation of the individual alga, and (b) direct secondary factors that directly affect the population density and, therefore, the total production which is possible in a given period of time.
Direct Primary Factors of Reproduction and Growth
The Energy Factors. In the presence of carbon dioxide and water, plants are able by virtue of their pigments to intercept the sun's radiant energy required by them in the manufacture of compounds of carbon. The chemical change is expressed in simple form by the equation 6CO2 + 6H2O → C6H12O6 + 6O2. This process of carbon assimilation is an endothermic reaction, wherein the energy absorbed is derived from the light and the resultant product contains more energy than was present in the reactants CO2 and H2O. This energy, stored in the complex organic substance of the plant, becomes the source of chemical energy for life processes, directly for those of the plant and of herbivorous animals and indirectly for those of carnivorous animals, and also for the heterotrophic bacteria and other saprophytic forms.
It is important to note that the by-product of carbon assimilation is free oxygen. The process therefore becomes a source of an appreciable portion of the dissolved oxygen in the water and, consequently, is of great moment in the general metabolism of the sea, in the respiration of organisms, and in oxidation of organic and inorganic substances.
Since oxygen is always used in the respiration of the algae, the free supply dissolved in the water is drawn upon for metabolic processes during periods of low light intensity or when the plants have sunk to a depth at which the light intensity is below the minimum required for oxygen production by photosynthesis to balance oxygen consumption by respiration. The depth where light intensity is just sufficient to bring about this balance is known as the compensation depth (cf. p. 779).
Factors of Food Supply. The utilization of carbon dioxide is directly associated with the process of photosynthesis and is briefly discussed above. It is sufficiently abundant in the sea at all times, either as a dissolved gas or as a fixed constituent of the bicarbonates, to meet the requirements of the plants; it is, therefore, apparently never a limiting factor in phytoplankton production in the sea, for as rapidly as it is consumed by the plants a supply becomes available by the hydrolysis of the bicarbonates (p. 192). It has been estimated by Moore (in John-stone, Scott, and Chadwick, 1924) that in the Irish Sea 20,000 to 30,000 tons of carbon dioxide per cubic mile of sea water are passed through the biological cycle annually.
Although carbon dioxide cannot be considered a limiting factor in plant production, it is an item of far-reaching biological significance. In the final analysis the carbon compounds of all living creatures of the sea, from protozoa to the higher animals, come directly from the carbon dioxide in the sea.
Under dissolved nutrient salts we include the mineral salts that have been shown to influence and at times limit or control the production of phytoplankton, namely, the nitrogen and phosphorus, and also, to a more uncertain degree, iron and other trace elements. The control of growth is expressed in Liebig's law of the minimum, which states that growth is limited by the factor that is present in minimal quantity.
In the early studies of phytoplankton, Brandt (1899) suggested that diatom growth must be regulated by the nutrient substances that the diatoms utilize in the lighted waters of the sea. He anticipated the importance of compounds of nitrogen and phosphorus as substances which, according to Liebig's law, might be limiting factors. With the improvements of analytical methods by Atkins and Harvey, it has been shown beyond doubt that Brandt's theory was correct. Various investigators have demonstrated that phytoplankton organisms may assimilate different compounds of nitrogen (cf. p. 915), not only nitrates but also nitrites and ammonia (Schreiber, 1927, Braarud and Föyn, 1931, Harvey, 1933, ZoBell, 1935). Of these, nitrates are the most important, and in waters of temperate and arctic latitudes they accumulate during the winter and form the main nitrogen supply for spring diatom growth (Cooper, 1937).
The utilization of nitrates and phosphates in the synthesis of organic substance proceeds at an approximately parallel rate so that, although both may become markedly reduced by plants, the ratio of the two approaches fifteen atoms of nitrogen to one atom of phosphorus, with some deviation called the “anomaly of the nitrate-phosphate ratio,” perhaps dependent upon stages in the nitrogen cycle, a more rapid bacterial regeneration of phosphates, and the history of the particular body of water. In keeping with this there is an agreement in the ratio
Although the two elements discussed above have been shown to be vital to plant production, yet in nature the diatom population has been known to decrease while these nutrients were still present in sufficient quantity to support production. In these instances the limiting factors must be looked for elsewhere.
Gran (1931) believed that low concentrations or lack of iron might be a factor in limiting plant growth at times, especially for neritic species. From experiments, this seems probable (Harvey, 1937), and Thompson and Bremner (1935) have recorded a reduction in the quantity of iron in sea water coincident with heavy diatom production.
There are still other elements that occur in minute quantities in sea water or as traces in the analysis of plants, but since the status of these in the economy of the plant is as yet uncertain, they will not be dealt with in this general survey. Manganese, for example, has been shown to influence the growth of the diatom Ditylum (Harvey, 1939).
Silicates, used in the formation of the siliceous shells of diatoms and of certain other organisms, show seasonal fluctuations and also vertical distribution correlated with phytoplankton activity (Atkins, 1926, Harvey, 1928). Yet there is no evidence that reduction of silicon ever becomes a limiting factor. However, data from areas especially rich in silicon show a degree of utilization of this element by diatoms that would exceed the total supply available if applied to areas of low silicon (p. 261).
Accessory Growth Factors. It is becoming increasingly evident that the nutritional requirements for a lively diatom growth include substances not referred to in the above consideration. In experimental cultures utilizing artificial sea water, Allen (1914) found that the diatom Thalassiosira gravida would not grow unless small quantities of natural sea water or extracts of the marine alga Ulva were added. The accessory growth substance added with the sea water and the alga is not known. More recently Harvey (1939) has shown that even natural sea water enriched with nitrate, phosphate, and iron may lack the growth substances necessary for the continuous production of the marine diatom Ditylum brightwelli. The experiment indicates also that there are complementary substances, neither of which function well without the other. Some diatoms, for example Nitzschia closterium, apparently do not need the accessory substances although their presence stimulates growth. It is thought that diatoms like Nitzschia may be able by their own activity to make the organic accessory required. The accessory substances are
The above will serve to illustrate in part the complex problems involved in the explanation of phytoplankton nutrition.
Factors Influencing Metabolism. The rate of metabolism is much accelerated with rise in temperature. According to van't Hoff's law the increase is two to three times for each 10° rise in temperature within favorable limits. Very little is known regarding the optimum temperature conditions for the various phytoplankton species. However, it is known that various species may be attuned to a given temperature range. The species with common temperature requirements constitute biological groups which, if cold-water loving, may thrive in the Arctic in summer and in lower latitudes only during colder seasons. The growing season for a cold-water group may thus shift toward the Equator as winter approaches, and that for a warm-water group poleward with the approach of summer.
The salinity of the water is important in maintaining the proper osmotic relationship between the protoplasm of the organism and the water. The extent to which osmotic relationships can vary is dependent upon the species. Very little study has been made on the osmotic relations of diatoms, but the diatom Ditylum apparently does not act as an ordinary osmotic system (Gross, 1940). Some diatoms, especially neritic forms, have a tolerance for a rather wide range of salinity and are known as euryhaline forms, while the oceanic forms are usually stenohaline, that is, capable of enduring only small changes in salinity. Changes in this factor operative mainly in estuarine or in inshore situations. The variations in salinity operate mainly as a selective agency determining the composition or types of species that make up the population rather than on the fertility of the region.
The hydrogen-ion concentration or pH of sea water is generally maintained within narrow limits owing to the buffer effect of the dissolved salts (p. 202), hence its range of variations at most, perhaps, has only a moderate influence as a limiting factor for phytoplankton. However, during periods of high diatom production a marked rise in pH does occur as a result of the rapid utilization of carbonic acid by the plants during photosynthesis. On such occasions an excessive rise might tend to act as a natural check on further proliferation of some species, although this has not been shown for diatoms. Gail (1920) found that growth of the brown benthic alga Fucus evanescens is much inhibited at the high pH
Direct and Indirect Secondary Factors Influencing Population Density
We have already described the methods of reproduction characteristic of the plankton algae. These methods, which involve mainly simple cell division, are highly efficient and under optimal nutritional and metabolic conditions result in building up by geometric progression an excessively dense population. Any factor, then, that disturbs this progression has a far-reaching effect on the total numbers that can be produced in a given period of time.
By way of illustration, Braarud shows in a hypothetical example the difference between two identical populations of 500 cells per liter after nine cell divisions, when one population suffers no loss between successive cell divisions, while the other is assumed to experience a net loss of 10 per cent between the successive divisions. The undisturbed population would have increased at the end of the given time to 256,000 cells per liter, whereas the other at the same time would have only 99,159 cells per liter. The total withdrawal in cells would be only 12,343, but these plus their potentiality to produce yet other cells in the given time would amount to 144,498 cells per liter, in other words, the difference in increase between the two initial populations of 500 cells per liter at the end of nine cell divisions.
Just how much time would elapse during nine cell divisions in nature is dependent upon the living conditions and the species involved. The rate of division is dependent, of course, upon the direct primary factors mentioned above. In culture experiments (Gran, 1933) in the sea at Woods Hole it was found that population increases corresponded to seven divisions in three days for Chaetoceros compressus, six divisions in five days for the oceanic species Rhizosolenia alata, and five to six divisions in three days for other species. It has been estimated by Harvey et al (1935) that diatoms divide at a rate of once each 18 to 36 hours.
In nature, the number of cells lost between successive divisions would not remain constant even when resulting from consumption by a uniform population of filter-feeding animals, for there is some evidence that the rate at which these animals filter water remains the same whether or not the water is thinly or thickly populated with plankton food (p. 901). Harvey et al also show that during periods of abundant food the grazing plankton animals consume more than they are able to digest and void pellets of only partially digested diatoms.
Consumption. Since the phytoplankton is the main pasturage of the sea, it is very heavily drawn upon by the countless numbers of herbivorous plankton animals living within or periodically invading the euphotic zone. This has long been recognized as a foregone conclusion (Hensen, 1887, Lohmann, 1908),
The planktonic copepods are doubtless the chief diatom grazers, and due to their vast numbers they must exact an exceedingly heavy toll. In examining the digestive tracts of copepods, Dakin (1908) found that diatoms were the most abundant organisms present, especially species of Thalassiosira and Coscinodiscus. Species of Peridinium were next in importance. Esterly (1916), in direct examination, also found evidence of phytoplankton grazing, there being included in the digestive tract diatoms, dinoflagellates, and coccolithophores. Lebour (1922), Marshall (1924), and others have also shown that diatoms are important in the diet of copepods.
In addition to the copepods there are also large numbers of other small animals, especially the larval stages of benthic invertebrates, that graze directly upon the phytoplankton. For example, larval annelids are voracious feeders and, when abundant, may diminish materially the diatom population. Lohmann, working at Kiel, calculated that during the course of a year the increase in volume of pelagic plants was 30 per cent daily. Hence, that fraction of the plant population could be consumed daily by grazers without endangering the plant stock, if no other loss occurred.
The unevenness of phytoplankton distribution both in space (horizontally and vertically) and in time may reflect relative intensity of grazing rather than control through nutrient or physical conditions of the water, and actually more organic production may have taken place in areas only moderately rich in plants at any given time (p. 901).
Sinking. Even though diatoms are especially adapted to a floating existence, the suspensory organs and analogous structures usually operate only to retard sinking and not as a complete counter to the constant pull of gravity. Therefore, many individuals of the population tend to sink below the euphotic zone and may be considered as being withdrawn from the reproducing population as completely as if consumed by animals in the euphotic zone, unless before death they are fortuitously returned to the lighted waters by ascending water movements. According to the definition of compensation values (pp. 778 and 779), when the depth to which the plants have sunk is characterized by light with intensity below the “compensation point,” theoretically they would be considered as having become, at least temporarily, nonproducers for the twenty-four hours considered. It is probable, however, that the slowly sinking specimens have an adaptability enabling them to carry on photosynthesis sufficiently to reproduce slowly at a lower illumination than is indicated for experimental cultures at the compensation point. Harvey (1939) found a “physiological state” in Ditylum which indicated an adaptability to
In connection with the velocity of sinking, temperature becomes an important indirect factor influencing production owing to its marked effect on viscosity. A marked discontinuity layer, providing a sharp temperature decrease within the euphotia layer, is an important feature in maintaining the plants in the productive layer, since viscosity is inversely proportional to temperature and the rate of sinking is inversely proportional to viscosity.
Vertical Transport. Some of the points discussed in relation to sinking will be equally applicable in the factor of vertical transport. We learn from the discussion of turbulence (p. 471) that water particles within a water mass are not at rest but may be shifted in various directions. Passive microscopic objects, such as diatoms, caught in these shifts are transported along with the water. But as far as transport is concerned, we are interested here mainly in the vertical component of turbulence. If this vertical component is confined to the transportation of particles up or down within the euphotic layer, its effect on the production will be of no great significance, although it is of importance in maintaining a “seed” supply in the euphotic layer. If, however, the movement involves a transport of water from the productive layer downward into darker layers, it will have a deleterious effect on production in so far as it carries with it a certain portion of the phytoplankton population. This deleterious effect may be partly compensated for by a return of normally sinking plants to the lighted zone by ascending currents, but the time spent below optimum light conditions must constitute a loss.
Indirect Factors. The indirect factors of productivity also belong to the secondary group and operate simultaneously with them, in most instances also with the direct primary factors. Temperature, for instance, modifies the rate of metabolism, while at the same time it regulates the viscosity, and the vertical temperature gradient tends to reduce active turbulence. On the other hand, turbulence, upwelling, or convection currents are essential to the return of nutrients to the euphotic layer; thus the effect on the population is very complicated.
Among the more obvious of indirect factors of productivity may be listed water movements of various kinds, stability of waters within the euphotic layer, discharge of rivers, meteorological conditions, bathymetric conditions, and geographic position. The separate indirect factors exert their influence on productivity through the effect on the direct factors, and also on the other indirect factors. As a result, a complex of forces is constantly at play to increase or to decrease the total production during shorter or longer periods of time.
FACTORS OF PHYTOPLANKTON PRODUCTION: II
The relation of plants to the physical-chemical and biological properties has been touched upon in the foregoing discussion outlining the factors that control phytoplankton production. It remains to enlarge upon and examine more closely how some of these factors operate in nature. This examination will be organized in reference to (a) photosynthesis of phytoplankton, (b) plant nutrients and vertical circulation of water, (c) horizontal ocean currents, and (d) temperature.
Photosynthesis of Phytoplankton
The intensity of light decreases gradually with depth; yet for the purpose of biological investigations it is convenient to consider the sea as divided vertically into three zones with respect to the amount of light that is present. The depths given are approximations and vary with latitude and season.
The euphotic zone, which is abundantly supplied with light sufficient for the photosynthetic processes of plants. This zone extends from the surface to 80 or more meters. All of the eulittoral zone lies within this layer.
The disphotic zone, which is only dimly lighted and extends from about 80 to 200 or more meters. No effective plant production can take place in this zone, and the plants found here have mostly sunk from the layer above.
The aphotic zone, the lightless region below the disphotic zone. In the deep sea it is a very thick layer in which no plants are produced and the animal life consists only of carnivores and detritus feeders.
Until the relationship between rate of photosynthesis and light intensity at different wave lengths is more thoroughly understood than at present, it will be necessary to restrict discussion to the relationship between rate of photosynthesis and total light intensity in the sea as expressed in energy units or in units of illumination.
When using energy units the light intensity is expressed in ergs per unit surface and unit time, for example, ergs/cm2/sec; or in gram calories or joules per unit surface and unit time (1 g cal = 4.18 × 107 ergs, 1 joule = 107 ergs). The penetration of radiant energy in the sea was discussed in chapter IV (p. 104). It was stated there that no direct measurements are available of the energy which under different conditions reaches different depths below the surface, but that these amounts could be computed from observations of the extinction coefficients for radiation of different wave lengths and from the intensity of the radiation of stated wave lengths reaching the sea surface (table 27, figs. 21, 22). In all experiments in which the intensity of light has been examined in conjunction with studies of photosynthesis, the energy at different levels has
In a number of published reports on photosynthesis in the sea, light intensity is expressed in units of illumination, that is, in units based on the power of light to produce visual sensation. Illumination units such as 1 meter-candle = 1 lux = 0.093 foot-candle have been widely used, and it is therefore necessary to explain their relation to the energy units. Because the unit of illumination is directly based on the reaction of the human eye, a mechanical equivalent of the illumination unit can be established only at a specified wave length. At moderate illumination the maximum sensitivity of the human eye lies at a wave length of 0.56 μ, and at this wave length the “luminous equivalent” has been established (Smithsonian Tables, 1933) at
There exists no simple relation, however, between the total energy of radiation of different wave lengths and the corresponding illumination in luxes, the reason being that the human eye is not at all sensitive to infrared radiation and is only slightly sensitive to radiation in the violet part of the spectrum. More than half of the radiation from sun and sky which reaches the sea surface falls in the infrared, and the eye is not very sensitive to a great portion of the remaining half, so that the luminous equivalent as defined above has to be multiplied by a factor of about 0.15 in order to give the illumination in luxes corresponding to a given intensity of the radiation in energy units. In the sea the infrared part of the radiation is absorbed in the upper few centimeters and, owing to the selective absorption in the visible part of the spectrum, the energy which penetrates to greater depths becomes more and more concentrated at wave lengths to which the human eye has its greatest sensitivity. Consequently, the factor by which the luminous equivalent must be multiplied in order to give the illumination in luxes increases with depth, reaching a value of approximately 0.75 at depths where the incident energy is reduced to 1 g cal/cm2/hour or less.It is not possible to give any table by means of which the energy reaching a certain depth can be converted into units of illumination, because the conversion factors will depend upon the quality of the light. Thus, in turbid coastal water and in the clearest oceanic water the same amount of energy is found at the depths of 10 and 100 m, respectively (table 27), but at 10 m in the coastal water the maximum intensity lies
Table 92 has been prepared in order to show approximately corresponding values of energy and illumination at different depths and in different types of water. For the sake of simplicity the intensity of the radiation penetrating the surface has been selected at 100 g cal/cm2/hour, corresponding approximately to the radiation from sun and sky with the sun at zenith. The illumination at the surface is then about 120,000 luxes. The illumination decreases more slowly with depth than does the energy, but it is realized that the values in the table do not correspond exactly to each other. They give only the approximate relationship and can serve in obtaining a rough estimate of the energy if the illumination is stated, or vice versa.
Depth (m) | Energy (gm cal/cm2/hour) | Illumination (luxes) | ||||||
---|---|---|---|---|---|---|---|---|
Oceanic water | Coastal water | Oceanic water | Coastal water | |||||
Clearest | Average | Average | Turbid | Clearest | Average | Average | Turbid | |
10 | 16.1 | 9.50 | 1.21 | 0.449 | 65,000 | 42,000 | 6800 | 2600 |
20 | 9.35 | 3.72 | 0.064 | 0.012 | 42,000 | 20,000 | 380 | 70 |
50 | 2.69 | 0.311 | 14,000 | 1,800 | ||||
100 | 0.452 | 0.0057 | 2,500 | 33 | ||||
150 | 0.076 | 440 |
The effect of light (somewhat modified by the effect of temperature) on photosynthesis can be illustrated by an experiment in which a series of suitable clear glass bottles are filled with sea water containing a substantial phytoplankton population and are submerged in the day time at various depths in the euphotic zone. It will be found that at a few meters below the surface the oxygen content of the water will increase as a result of the photosynthetic activities of the plants within the bottles.
Depth range (m) | Temperature (°C) | Condition of bottles | Oxygen after exposure (ml/L) | Change in oxygen (ml/L) | Oxygen produced (ml/L) |
---|---|---|---|---|---|
0 | 10.75 | covered | 7.11 | −0.71 | |
exposed | 9.42 | +1.60 | +2.31 | ||
exposed | 9.44 | +1.62 | +2.33 | ||
10–8 | 10.60 | covered | 7.32 | −0.50 | |
exposed | 8.40 | +0.58 | +1.08 | ||
exposed | 8.44 | +0.62 | +1.12 | ||
20–16 | 10.0 | covered | 7.42 | −0.40 | |
exposed | 8.07 | +0.25 | +0.65 | ||
exposed | 8.02 | +0.20 | +0.60 | ||
30–24 | 6–9 | covered | 7.61 | −0.21 | |
exposedxs | 7.81 | −0.01 | +0.20 | ||
exposed | 7.72 | −0.05 | +0.16 | ||
40–32 | 5–6 | covered | 7.54 | −0.28 | |
exposed | 7.66 | −0.16 | +0.12 | ||
exposed | 7.71 | −0.11 | +0.17 | ||
50–40 | 4–5 | covered | 7.61 | −0.21 | |
exposed | 7.59 | −0.22 | +0.01 | ||
exposed | 7.64 | −0.18 | −0.03 |
When the temperature decreases with increasing depth, the rate of respiration decreases. As long as the light intensity is so high that it does not limit the rate of photosynthesis, this rate also falls somewhat with falling temperature, but at low light intensities the effect of temperature
The photosynthesis depends on the light intensity, and the light intensity at which oxygen production and oxygen utilization are equal is therefore called the compensation point, or the compensation light intensity. The illumination at this point is “the minimum intensity at which the plant could survive in nature and [is] still too low for any crop increase” (Jenkin, 1937). The more pronounced effect of lowered temperature on the rate of respiration as compared with the effect on the rate of photosynthesis leads to a slight lowering of the compensation point with decreasing temperature, at least in certain higher algae (Spoehr, 1926).
It is obvious that the compensation point is determined by physiological characteristics of the plants and may, therefore, be somewhat different for different species, just as the optimum light intensity is not the same for all species. The compensation point is independent of the time during which photosynthesis and respiration have been measured if the oxygen production per unit time remains proportional to the light intensity and the oxygen consumption per unit time remains constant. On these assumptions, the oxygen production dP in the short time interval dt equals aIdt, where a is a constant and I is the light intensity, and the oxygen consumption dR equals bdt where b is another constant. The compensation point, Ic, is defined by
The values of oxygen production P and consumption R in the time T are The average light intensity in the time T is Therefore, if P = R, it follows thatThe two factors a and b which have been introduced here are in general not determined, but measurements of the difference between production and consumption are made at different light intensities and the compensation point is found by interpolation. Since the compensation point depends upon the ratio b/a, it follows that it depends on temperature if photosynthesis and respiration are influenced differently by the temperature.
The depth at which the compensation point is found is called the compensation depth. This depth evidently varies with the season of the year, the time of day, the cloudiness, and the character of the water. In the experiment quoted in table 93, the compensation depth lies between 27 m and 44 m. For a given locality one can introduce such terms as daily compensation depth with a clear sky, daily compensation depth with an overcast sky, average annual compensation depth, and so on. With a clear sky the compensation depth will in a given locality reach its maximum value at noon and will rise to the surface shortly before sunset. The average daily compensation depth is less than the compensation depth at noon, except at the North Pole.
The diatoms and many other algae thrive best in somewhat subdued light, but the optimum light intensity is not the same for all species. For example, Schreiber (1927) found the optimum for the diatom Biddulphia mobiliensis to be 1600 luxes, and for the green flagellate Carteria sp. over 3200 luxes. Some diatoms are able to grow in much reduced light. This has been shown in nature by Vanhoeffen for arctic species found growing under the ice in March. From Schreiber's results with Biddulphia we learn that high illumination does not necessarily increase the rate of photosynthesis, since the rate of multiplication was proportional to light intensity only at the intermediate illuminations 200 to 400 luxes. That this is quite generally true is indicated by the habit exhibited by many diatoms of grouping the otherwise scattered chromatophores into knots when exposed to strong light. In this way they afford mutual protection during such periods of unusual exposure as might occur when being swept by ascending currents to the very surface on bright days. This condition whereby the chromatophores are concentrated or collected into a group is known as a systrophe, and was described by Schimper (Karsten, 1905). For the diatom Coscinodiscus excentricus this has been found to occur at a light intensity of about 9.6 g cal/cm2/hour, p. 781. That diatom photosynthesis falls off
Assimilation experiments with raw plankton samples can be carried on only if the diatom population is sufficiently dense that the metabolic activities provide differences in oxygen content and pH values above the limits of experimental error of the method. Experiments with persistent cultures have the advantage that they make possible field-assimilation experiments even at seasons when in nature the phytoplankton is at a minimum. The absence of small planktonic animals and the possible control of bacterial populations in such cultures eliminates a source of error in computing oxygen utilization. On the other hand, the diatoms in persistent cultures may represent selective species with slightly different light optima and may therefore not be typical of the mixed population of raw cultures.
The considerable variation found for the depth of compensation of oxygen production and utilization by phytoplankton results from variations in latitude, season, weather, and turbidity, all of which influence the depth of submarine illumination. Theoretically, it should be possible to determine the depth of optimum diatom production for a given area during the growing season by a study of the vertical distribution of
Not only is the quantity of light a factor to be considered, but so also is the quality. It is well known that as sunlight enters the sea the intensity is reduced, but there is moreover a differential absorption, certain light rays being absorbed before others. The red component of light is removed in the first few meters of water, while the blue light penetrates to much greater depths (p. 106). Therefore, diatoms in nature have their greatest growth at depths where the red component is much reduced; rich growths are found at depths (15 to 20 m) where only blue and green light prevail. A realization of this has led to experiments on the effect of light intensities selectively reduced.
In experimental cultures, using Nitzschia closterium, a diatom much used in culture experiments, Stanbury (1931) concluded that the precise wave length is not so important as the amount of energy transmitted.
Assuming that the total energy in all wave lengths within the visible spectrum provides the best measure of available energy for photosynthesis of diatoms, Jenkin (1937), working in the English Channel, computed the available energy in joules or gram calories at various depths and correlated these values with the amount of oxygen produced by Coscinodiscus excentricus in submerged experimental bottles at the various depths tested. The results indicate that with an energy flux less than 7.5 joules, or 1.8 g cal/cm2/hour, the oxygen production is directly proportional to the energy and the utilization of the available energy is about 7 per cent. With an energy flux greater than the above, the photosynthesis is gradually inhibited. Systrophe takes place at an
In certain sessile shallow-water diatoms adapted to a depth of only a few centimeters the maximum oxygen production occurs at higher energy values, that is, 60 g cal/cm2/hour or 80,000 luxes (Curtis and Juday, 1937).
Diatoms tend to show chromatic adaptation when grown under different components of light, assuming colors that are complementary to those under which they are grown. They thus tend to become yellow-green when grown in red and yellow light, and dark brown in green and blue light. For benthic green algae the red component of light is essential to healthy growth, while brown algae and, especially, red algae characteristic of deep water are adapted to carry on photosynthesis with the red component absent or reduced. We have seen also (p. 295) that, in keeping with this, the order of vertical distribution of the benthic marine algae is greens, browns, and reds, from shallow to deeper water. The quantity of chlorophyll present in these classes of algae is also diminished in the same order (Lubimenko and Tikhouskaia, 1928). The depth at which these benthic algae can grow is also directly correlated with the depth of submarine illumination. In northern latitudes the maximum depth is about 40 to 50 m, whereas in the clear waters of the Mediterranean it is said to be over 100 m. It has been suggested (Klugh, 1930) that certain pigments present in plants growing some distance below the surface may act as photosensitizers to the chlorophyll, enabling it to function with less light intensity.
Plant Nutrients and Vertical Circulation of Water
The inorganic substances required by plants are commonly spoken of as “plant nutrients.” These constitute the raw materials or building blocks out of which the plants, with the aid of sunlight, build up organic compounds entering into their cell structure. The growing plants can obtain these raw materials only from the sea water in which they live. This must therefore be looked upon as a dilute nutrient medium containing all of the elements necessary for plant growth. The concentrations of the nutrient salts are remarkably small; yet a concentration of 1 mg of phosphorus to 10001 of water, for example, is sufficient to produce a vigorous growth of diatoms if other factors are favorable. This is facilitated partly by the very minute size of most phytoplankton organisms and may be illustrated by comparing one plant 1 mm3 in size with a
The mineralized nutrient elements are not uniformly distributed in the sea, however, and they undergo cycles during which there are periods of delay between the available mineralized state and the unavailable organically bound state of the nutrients. This leads to fluctuations in the intensity of plant production both in space and in time. The production of marine phytoplankton is nature's greatest demonstration of hydroponics, or water culture. The operation of the whole system in detail in the sea is different in many respects, however, from the operation of experimental hydroponic tanks. For example, there is a difference in the population; as we have learned, the floating plants of the sea are not specialized with aerial portions for utilization of CO2 and elimination of O2; and roots are not provided for intake of mineral nutrients; neither are there elaborate vascular systems to transport fluids and food. All of the metabolic processes of the marine phytoplankton are carried on within the individual cells which are always submerged beneath the water surface. This universality means that the whole plant must receive light, and the specializations, as already discussed, are along lines designed to maintain the plants in the upper lighted portions of the sea. In the sea the mineral nutrients are not added from without as needed; they have accumulated from the land over eons of time and are stored mainly in the deeper dark-water layers where they are not accessible to autotrophic plants and from whence they must be transported by water movements to replenish the supply periodically exhausted in the productive lighted zone. For discussion of the forces causing this transport see chapter XIII.
It is well known that with return of sunshine and increased temperatures during spring in the temperate and higher latitudes there is a phenomenal outburst of phytoplankton growth in the surface waters. Even the most causal observer is impressed with the brown-green color that is imparted to the waters of coastal areas and over banks during these periods of diatom “bloom.” The production may go on at varying intensities during the summer and frequently becomes augmented again in the autumn. But during the winter months, and sometimes at other seasons as well, there is a dearth of plants and the waters again become deeper blue in color. Similar conspicuous changes in water color occur when one leaves the rich coastal waters and proceeds to the barren open
Brandt's theory that phosphates and nitrates may constitute limiting factors in phytoplankton production has found proof in investigations of subsequent workers (Marshall and Orr, 1927, Schreiber, 1927, Gran, 1930, Hentschel and Wattenberg, 1930, and others). At the initiation of vernal phytoplankton production these salts are relatively abundant in the euphotic layer, but as production proceeds the quantity gradually falls off until it can no longer maintain a large population, and the organisms diminish in number or disappear from the water.
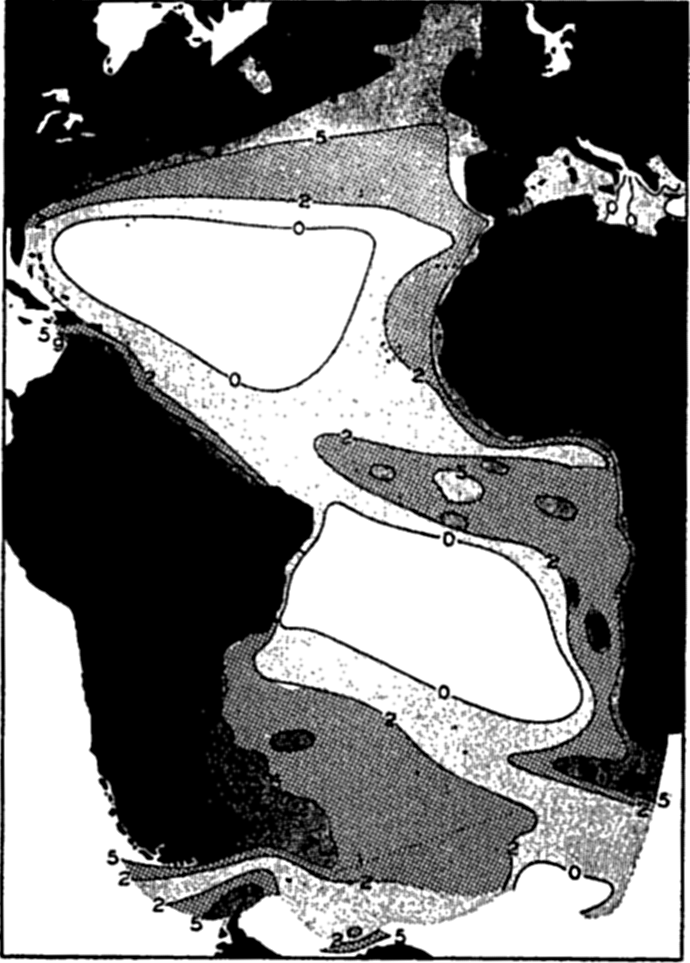
Color of the sea as indicated in per cent of yellow according to the Forel scale (from Schott).
[Full Size]
Here Nathansohn's theory forms a complement to Brandt's theory in the explanation of irregularities in distribution and periodic fluctuations in phytoplankton production. (See p. 901 for control through organic factors.) Nathansohn (1906) suggested that the nutrient salts must disappear from the lighted surface layers owing to the fact that they are consumed by the plants and that they must therefore accumulate in the deep sea through sinking of living or dead bodies, both plant and animal, constantly settling towards the bottom. The store of nutrients thus accumulated in the deep water through the dissolution of these bodies is, in time, returned to the euphotic layer through diffusion and vertical circulation of the water.
With improvements of methods in chemical analysis it has, indeed, been shown that usually phosphate concentrations do increase with
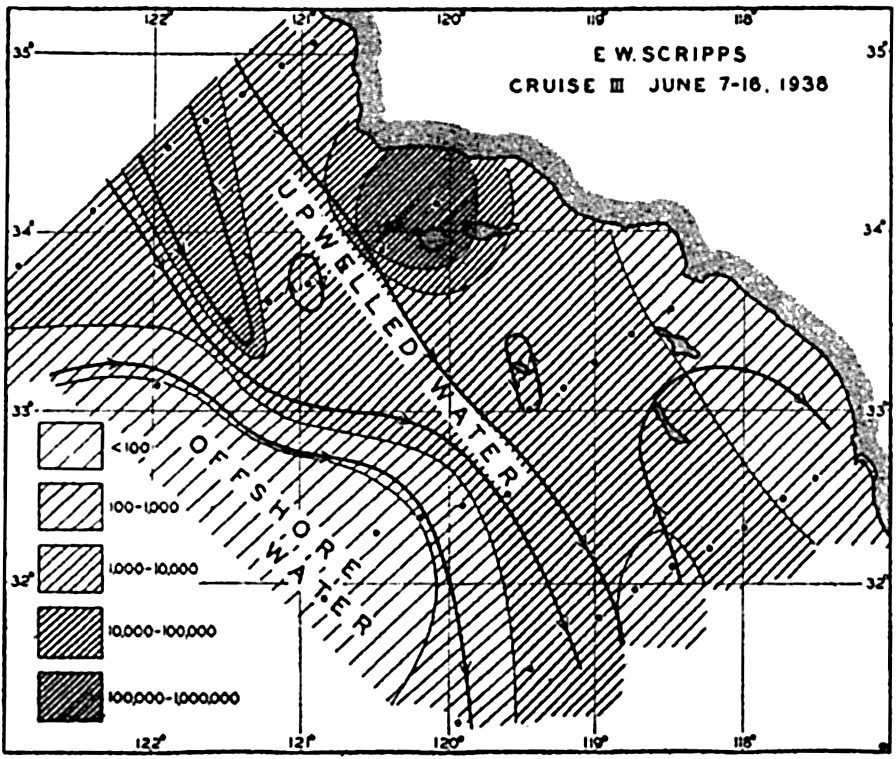
The average number of diatoms per liter between the surface and 60 or 70 m, off the California coast. Character of currents is indicated by heavy lines with arrows, and distribution of diatoms is indicated by hatching (modified from Sverdrup and Allen).
[Full Size]
Upwelling. The upwelling of subsurface water in which nutrient salts are brought back to the lighted layers is reflected in large diatom production along the California coast (Moberg, 1928, Sverdrup and Allen, 1939; fig. 215), along the western South American coast through the mechanism of the Peruvian Coastal Current (Gunther, 1936), in regions of divergence along the equatorial countercurrents, and along
Not all surface currents show this tendency to unusually rich fertility indicated by the above great coastal populations and the seaward tonguelike extensions. In seeking an explanation we find that the upwelling along the African coast has brought to the surface nutrient-rich water, fertilizing the illuminated euphotic layer. The plants respond with a luxuriant growth, which diminishes in intensity as the nutrients are gradually consumed by the population in the waters as they move to greater and greater distances away from the coast and from the regions of upwelling. Figure 217 shows how closely the distribution of phosphates coincides with the areas shown by direct examination of plankton catches to be fertile in phytoplankton.
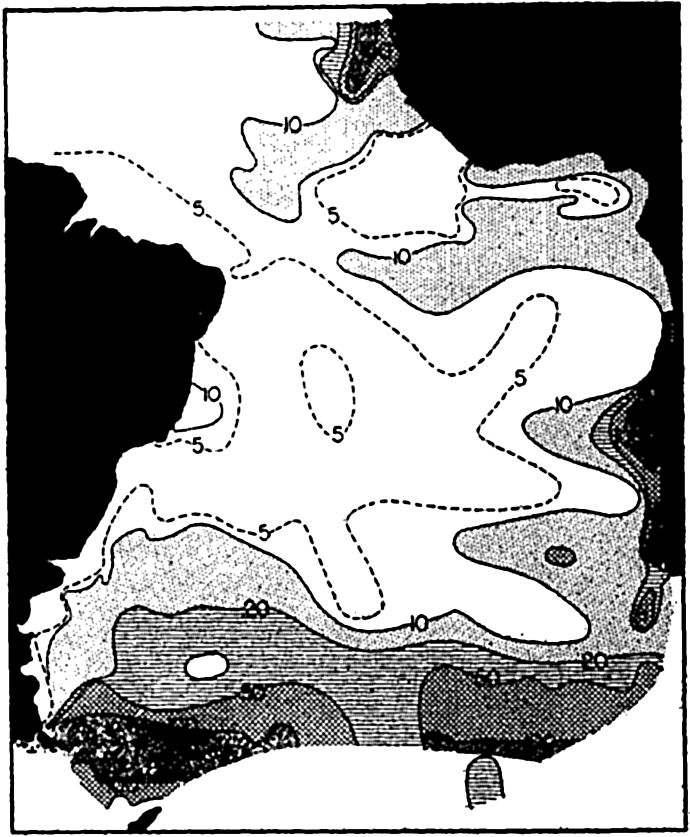
The concentration of total plankton (micro- and nannoplankton) in the South Atlantic, surface to 50 m. The numbers on the curves represent thousands of individuals per liter.
[Full Size]
The distribution of phosphates as shown in fig. 218, which represents a vertical section from the coast of Africa across the Atlantic to the coast of South America at lat. 9°S, also illustrates how nutrient-rich water is nearer the surface on approaching the African coast. The water with more than 1 μg-atom/L (30 mg/cm3) rises to within about 40 m of the surface, whereas on the South American coast water of nutrient concentration greater than this is not found above about 1000 m. It must be borne in mind that not only phosphates, but also other nutrients needed for plant life that have been regenerated in the deep waters from sinking bodies of organisms produced in the upper layers, are conveyed from the
Regions of Divergence and Convergence. Regions of divergence are defined as those regions where surface water masses of the ocean flow away from each other or away from the coast so that water from the deep must rise, as a feature of upwelling, to replace them. It appears that such areas, whether at the coast or in the open sea, are productive of phytoplankton; this condition is exemplified in the South Atlantic Congo tongue of cold water which extends far out to sea from the region of the Congo River (Hentschel, 1928). Conversely, regions of convergence are areas where surface waters meet and sink. Such areas are relatively barren of plants, the waters having been impoverished by earlier exhaustion of the nutrients.
The hydrographic features of these two types of water movements are discussed on p. 140, and graphically shown in fig. 198, p. 710, for a Carnegie section across the equatorial currents of the Pacific. Here are two parallel regions of divergence between which lies a region of convergence associated with the Equatorial Countercurrent. When the Carnegie plankton volumes from H. W. Graham's analysis (1941) are compared with the hydrography of this section, we find that the relative volumes of general plankton, which must reflect phytoplankton production, show greater concentrations at stations 157 and 151 which lie within the regions of divergence. This is brought out in fig. 219, where the upper diagram shows the relative plankton volumes (precipitated in special bottles) in centimeters per mile of haul, and the lower diagram shows the vertical water movements and selected isolines for phosphate. For greater detail, see fig. 198.
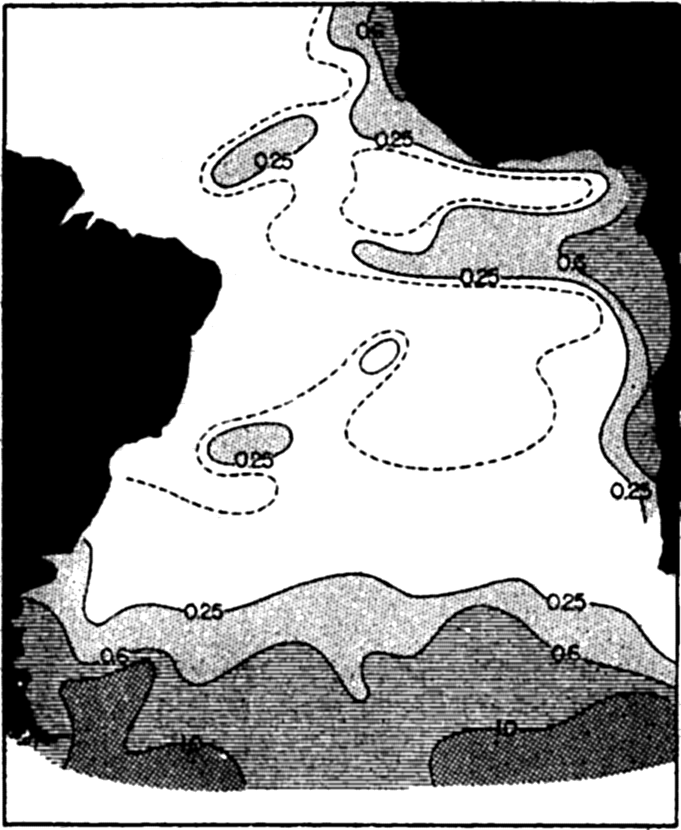
Horizontal distribution of phosphates in the South Atlantic: mg P2O5/m3 (from Wattenberg).
[Full Size]
Turbulence. The effect of renewal of plant nutrients from the deeper water as the result of turbulence and accompanying diffusion is demonstrated especially in such areas as the Bay of Fundy and Puget Sound, where strong tidal currents flowing over uneven bottom and through tortuous passages maintain a relatively homogeneous water
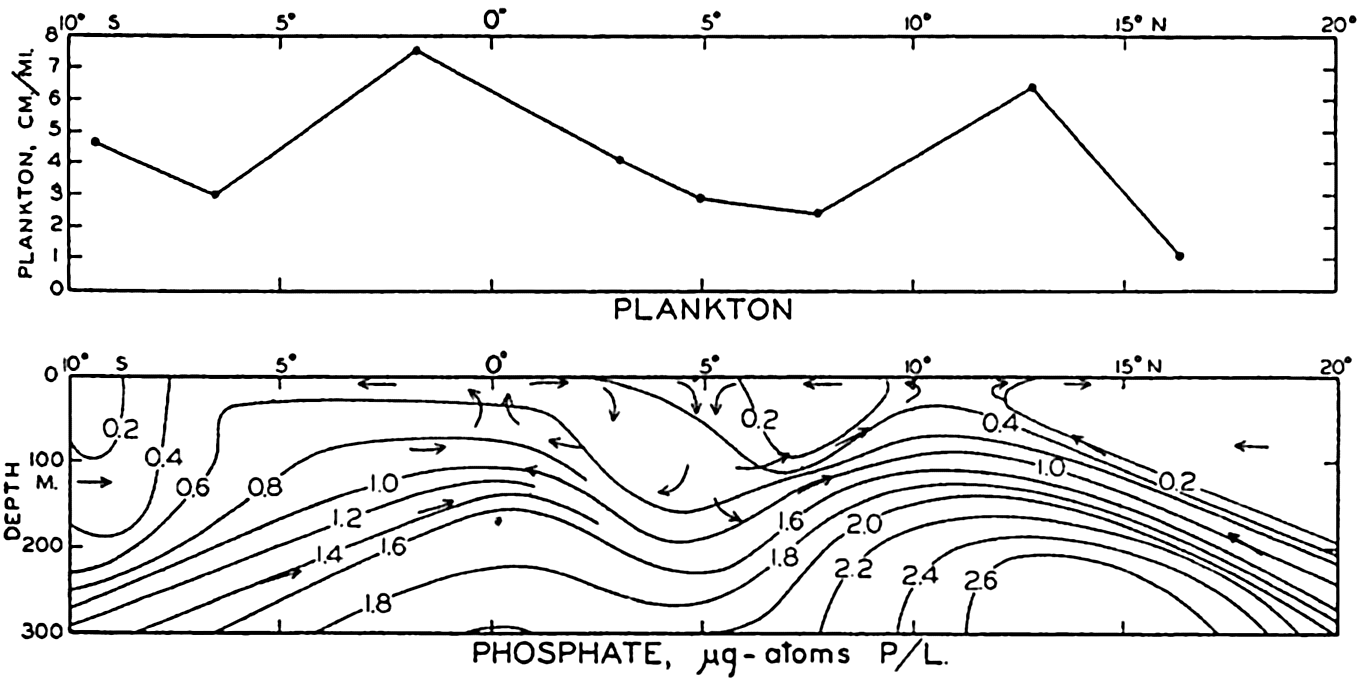
Upper: concentration of plankton in a section across the Pacific Equatorial Countercurrent. Lower: phosphate concentration for the same section.
[Full Size]
As far as renewal of plant nutrients is concerned, upwelling and turbulence are similar in that they are more or less continuous throughout the year with some variation resulting from periodic changes in the direction or intensity of the winds (cf. p. 725) or from stabilization resulting
Where turbulence and upwelling are sufficiently vigorous to involve the euphotic layer, there may always be present a ready supply of nutrients to support at least a moderate plankton production. Under such conditions the cessation of production must result from other factors than depletion of nutrients. During the winter season the controlling factors in some latitudes may be the reduction of light or temperature. The cessation of reproduction during a portion of the year may well represent a period of rest induced by a change in conditions, at least for neritic species.
Stabilization. During recent years there has been a realization that for maximum production a certain degree of vertical stability is required within the euphotic layer. In areas of excessive turbulence this stability is wanting, with the result that, although nutrients and other factors may be optimal, only a moderate production of phytoplankton takes place because the descending currents withdraw a portion of the diatom stock into the deeper water where there is not sufficient light for photosynthesis. Ascending currents may bring some of these back to depths of adequate light. The length of time spent below the euphotic layer would then determine the extent of loss, if any, to total production. The complex problem introduced by turbulence may be stated as mainly one of balancing the beneficial factor against the deleterious factor: ascending currents constantly conveying nutrients from deeper water against descending currents withdrawing a portion of the population to suboptimal lighting conditions. The depth of turbulence and the thickness of the euphotic layer are important in this connection. An example of the beneficial effect is seen when comparing areas in the Gulf of Maine and the Bay of Fundy (Bigelow, 1926, Gran and Braarud, 1935). In the open Gulf, marked stratification due to summer heating leads to the exhaustion of nutrients in the euphotic zone with a subsequent drop in phytoplankton production. Over Georges Bank, however, a fairly rich diatom plankton is maintained during the summer owing to the turbulence of water flowing over the bank.
The deleterious effect is illustrated in parts of the Bay of Fundy where, in June, surface areas rich in nutrients, 0.3 μg-atom/L PO4-P (10 mg PO4-P per m3) and up to 4 μg-atoms/L NO3-N (60 mg NO3-N per m3) at a depth of 1 m, resulting from turbulence, were nevertheless poor in plankton because of the combination of turbulence and low transparency. Diatoms were fewer than 500 cells per liter in the most affected areas. The largest populations were found in areas of moderate stratification. Two months later some stabilization had occurred with the advance of summer, but the most turbulent areas still showed high nutrients and a small population of about 7000 or fewer diatoms per
Convection. In regions where there are marked seasonal changes in temperatures between winter and summer, the changes are of great importance not only because of the effect of temperature directly upon the rate of metabolism but also because of its indirect effect in the renewal of nutrients to the surface layers. This method of renewal is characteristic of fresh-water lakes, but is also very important in the sea, especially in the higher latitudes. The phenomenon is, of course, dependent upon the surface waters cooling to a point where their density becomes sufficient to cause them to sink and be replaced by upward movement of lighter and incidentally nutrient-rich waters from below. Similar effects result from pronounced evaporation and must contribute essentially to the maintenance of phytoplankton in the open tropical seas and in isolated seas like the Mediterranean. In areas where vertical circulation results from convection currents, the biological implications are somewhat different than those resulting when nutrient renewal is brought about through the agency of upwelling or turbulence. Except as discussed later, renewal of surface waters by convection is a seasonal rather than a continuous process. The replenishment of elements essential to plants occurs during autumn and winter, with the result that the euphotic layer is richly fertilized in readiness for diatom growth as soon as sufficient sunshine becomes available in spring and as soon as other favorable living conditions are attained. A period of intense growth then ensues, resulting in depletion of nutrients, and since no general renewal can take place until autumn such areas typically experience a marked summer minimum in diatom production following the spring maximum. There is usually a secondary autumnal maximum before the gradually diminishing light reaches a point too low for effective photosynthesis. The regularity of this system may be disturbed by wind-induced turbulence sufficient to cause an upward transport of nutrientladen water from deeper layers, or by fertilization of the surface layers with nutrients brought down from land by rivers. Thus there may be alternating maxima and minima of greater or lesser duration and intensity throughout the whole growing season.
In boreal waters, when the spring production of diatoms has come to a low ebb owing to marked stratification and near exhaustion of nutrients, the role of organic production may be taken over in a diminished degree by the dinoflagellates, especially members of the genus Ceratium. These organisms, owing to their low nutrient requirements and slow rate of growth (daily increase under summer conditions of only 30 to 50 per cent as opposed to 360 per cent in the diatom Chaetoceros curvisetum) can develop at lower nutrient salt concentrations than can diatoms, and they may therefore continue to propagate in impoverished waters.
Horizontal Ocean Currents
As an outcome of the phytoplankton collections taken by the Challenger from the various seas of the world, it appeared that in general no essential differences existed between the pelagic flora of different seas; but later, as a result of the more detailed plankton work of the German Plankton Expedition, Schütt concluded that different ocean currents are inhabited by characteristic types of floating plants.
Cleve (1900) was of the opinion, after studying extensive plankton collections from many separate areas, that plankton species endemic to separate geographic areas (each with its own set of environmental conditions) are transported from these areas by water currents to remote regions, depending upon the hydrographic conditions prevailing. Accordingly, the procedure of investigation should be first, to determine the geographic distribution of the separate species and to learn the conditions of life required by the principal plankton associations. Then, according to the theory, the origin of various water masses could be determined by a biological analysis of the waters to ascertain the type of plankton organisms they support. Cleve recognized a number of plankton communities with dominant types characteristic of separate ocean regions wherein the water has certain uniform characteristics with respect to the physical characters, but the hope of utilizing the diatom plankton types as indicators of water origin has not been fully realized. The nature of the life history of diatom populations, their prompt response by increase or decrease to fluctuating conditions of nutrients and other variable environmental factors, must make them of less value as direct indicators of ocean currents than are the planktonic animals with longer, more tenacious lives. Certain species of Ceratium have proved of special value in characterizing water masses, for example, the fluctuating flow of Arctic and Atlantic waters around Newfoundland (p. 865).
The diatom plankton types of Cleve are of great significance, however, as biological types (p. 793), each with different requirements of life which make them respond in succession to changes of season as living conditions become favorable to their specific needs.
From the strictly biological aspect, we are especially interested in the dispersal of species that is brought about by means of ocean currents. The widespread distribution of most species is evidence in itself of the operation of this type of dispersal. Many species are common to widely separate areas where living conditions are similar during at least a portion of the year. The diatom Asterionella japonica was not recorded from the waters of Romsdalsfjord, Norway, during investigations covering the
It is not uncommon to find small numbers of neritic diatoms that have been carried far seaward with outgoing currents. They may even reproduce for a time by fission but they gradually become abnormal, with weakly silicified shells and diminutive in size; some may form resting spores which drift about. Just what are the oceanic factors that are unfavorable to neritic species is not clearly known. It may be a matter of nutrition or salinity, though, with respect to the latter, many neritic species are doubtless euryhaline.
The most popularly known drift of marine plants is that of the higher alga Sargassum. Although naturally an attached littoral plant, Sargassum may be torn from its moorings along the shore and float far to sea with the currents. Under these conditions it thrives vegetatively but is unable to reproduce except by fragmentation. Its tendency to accumulate in the slowly flowing cyclonic circulation in the North Atlantic has given the name Sargasso Sea to that relatively quiet halistatic area. Commonly, other littoral seaweeds and animals live attached to or among the branches and gas-filled floats of the Sargassum.
Temperature
Because of the close association of temperature with other factors, we have already had numerous occasions to refer to it in the course of discussion. It is certainly one of the most important of physical factors. However, it is not easy to generalize and designate any one factor as being more important than any other in a complex natural environment where all of the numerous known factors operate simultaneously. According to Liebig's law of the minimum, production is limited by the factor occurring in minimal quantity. This factor is obviously not the same for each circumstance. Hence, actually, the factor occurring in minimal quantity, whatever it may be, is temporarily the most important factor. It becomes the so-called “limiting factor” which has been defined as “any of the direct factors which is not optimal at the conditions present in a water mass, viz., without any change of the other factors.”
The rate of metabolism, hence the rate of growth and reproduction of any given species, is regulated by temperature. Just what will be the required range of temperature is dependent upon the species, but the warmest as well as the coldest waters of the sea are able, when other external factors are favorable, to produce a characteristic type of flora
April, 3°C | Thalassiosira nordenskioeldi |
Porosira glacialis | |
Chaetoceros diadema | |
May, 6°C | Chaetoceros debilis |
June, 9°C | Chaetoceros compressus |
August, 12°C | Chaetoceros constrictus |
Chaetoceros cinctus | |
Skeletonema costatum |
Neritic species of the higher latitudes are also durothermic with respect to low temperatures during resting stages. These resting stages are very resistant to extreme temperatures and have been found to be viable even though they have spent unfavorable periods frozen solidly in ice.
We may conclude, then, that one great influence of temperature is in determining the character, that is, the composition, of species that will develop in any one region at various periods of the year. In temperate latitudes it is possible, depending on season and other conditions, for both high-latitude and subtropical species to grow provided either that “seed” can be carried in by currents at the proper season or that durothermic resting stages can survive the “off” season and germinate again with return of the growing season.
In this way Cleve's geographical diatom types, namely, arctic, boreal, temperate, and tropical, are explained not as having been produced in and carried from these separate areas but rather as having their metabolic requirements adjusted to temperatures characterizing these regions. During seasons of unfavorable temperatures the stock of separate types is maintained as resting-spores or resistant individuals with potentialities of quick response upon the return of their specific temperature tolerance, the different species succeeding each other in order according to their requirements.
Perhaps one of the most far-reaching influences of temperature is indirect through its effect on the viscosity of the water. We have had frequent need to mention how vitally important it is that the open-sea plants be kept within the euphotic layer. The degree of viscosity or internal friction of the water is vital to passively floating organisms with a specific gravity greater than the water in which they live. Variations
Winter and summer variations which occur in the structure of certain species are attributed to the temperature variations of the seasons in areas where there is great temperature range, but temperature differences in higher and lower latitudes have the same effect, consequently summer forms of high-latitude species may occur in the warmer seas or vice versa. The structural differences are concerned mainly with adaptations leading to a better adjustment to the floating existence. The summer or warm-water forms have thinner shells and are of more slender build. A classical example is given in the diatom Rhizosolenia semispina (fig. 70), wherein a temperate form (semispina) and an arctic form (hebetata), once regarded as separate species, may originate one from the other or wherein opposite ends of a single cell may even show characteristics of both. The form hebetata is now regarded as a primitive resting stage of the form semispina.
In concluding this discussion it should be emphasized that even though the conditions with respect to light, temperature, nutrients, and so forth may be favorable to diatom production, yet no spontaneous population development can take place if previous conditions with respect to any factor have left the water sterile of vegetative plants or of resistant resting spores of species capable of taking advantage of the return of good conditions. This may explain in some instances the dearth of phytoplankton in offshore situations where the great depth of water prohibits the ready return of resting stages common to species of neritic waters. The phenomenal outbursts of diatoms in arctic regions coincident with the spring melting of ice have been explained as probably being associated with the rapid germination of spores that have been locked in the ice and are released through melting. It is known that these regions simulate biological conditions of coastal areas and the population is largely neritic. In investigating oceanographic conditions at a line of stations through Barents Sea from the coast northward to the Arctic ice, Kreps and Verjbinskaya (1930) found in spring that the early vernal flares of diatoms were confined to coastal waters and to waters near the Arctic ice, while between these and at some distance from
The stimulation of phytoplankton growth commonly observed to be coincident with the mixing of two bodies of water with different characteristics may also result from an inoculation of a sparse population living under poor conditions into more favorable waters which have lacked spores suitable to take advantage of the good physical-chemical environment.
Population control through biological factors, such as animal consumption, is discussed in chapter XVII.
Bibliography
Allen, E. J.1914.
“On the culture of the plankton diatom Thalassiosira gravida Cleve, in artificial sea water”
. Marine Biol. Assn. U. K., Jour., v. 10, p. 417–439, 1914. Plymouth.
Allen, E. J., and E. W. Nelson. 1910.
“On the artificial culture of marine plankton organisms”
. Marine Biol. Assn. U. K., Jour., v. 8, p. 421–474, 1910.
Allen, W. E.1936. Surface plankton diatoms in the North Pacific Ocean in 1934. Madroño, v. 3, p. 1–3, 1936.
Atkins, W. R. G.1926.
“A quantitative consideration of some factors concerned in plant growth in water”
. Conseil Perm. Internat. p. l'Explor. de la Mer, Jour. du Conseil, v. 1, p. 99–126, 1926.
Bigelow, Henry B.1926.
“Plankton of the offshore waters of the Gulf of Maine”
. U. S. Bur. Fisheries, Bull. 40, p. 5–487, 1926.
Braarud, T.1935. The Öst expedition to the Denmark Strait, 1929. II. The phytoplankton and its conditions of growth. Hvalradets Skrifter, no. 10, p. 5–144, 1935. Oslo.
Braarud, T., and B. Föyn. 1931.
“Beiträge zur Kenntnis des Stoffwechsels im Meer. Norske Vidensk”
. Akad., Avhandl., no. 14, 24 pp., 1931.
Brandt, K.1899. Ueber den Stoffwechsels im Meer. Komm. z. Wissensch. Untersuch. Deutschen Meere in Kiel und d. Biologischen Anstalt auf Helgoland. Wissensch. Meeresuntersuch., N.F., Abt. Kiel, Bd. 4, p. 213–230, 1899.
Clarke, G. L., and R. H. Ostler. 1934.
“The penetration of the blue and red components of daylight into Atlantic coastal waters and its relation to phytoplankton metabolism”
. Biol. Bull., v. 67, p. 59–75, 1934.
Cleve, P. T.1900. The seasonal distribution of Atlantic plankton organisms Göteborgs Kundl. Vet.-och Vitterhets Samhalles Handlingar, v. 17, 36 pp., 1900.
Cooper, L. H. N.1937.
“On the ratio of nitrogen to phosphorus in the sea”
. Marine Biol. Assn. U. K., Jour., v. 22, p. 177–183, 1937.
Curtis, J. T., and C. Juday. 1937.
“Photosynthesis of algae in Wisconsin Lakes. III. Observations in 1935”
. Intern. Rev. d. ges. Hydrobiol. u. Hydrogr., Bd. 35, p. 122–133, 1937.
Dakin, W. J.1908.
“Notes on the alimentary canal and food of the Copepoda”
. Intern. Rev. d. ges. Hydrobiol. u. Hydrogr., Bd. 1, p. 772–782, 1908.
Esterly, C. O.1916.
“The feeding habits and food of pelagic copepods and the question of nutrition by organic substances in solution in the water”
. Calif. Univ., Pub. in Zool., v. 16, p. 171–184, 1916.
Gaarder, T., and H. H. Gran. 1927.
“Investigation of the production of plankton in the Oslo Fjord. Conseil Perm”
. Intern. p. l'Explor. de la Mer, Rapports et Proc.-Verb., v. 42, 48 pp., 1927.
Gail, F. W.1920.
“Hydrogen ion concentration and other factors affecting the distribution of Fucus”
. Puget Sound Biol. Sta., Pub., v. 2, p. 287–304, 1920. (Univ. of Washington, Oceanographic Laboratories, Seattle.)
Graham, Herbert W.1941.
“Plankton production in relation to character of water in the open Pacific”
. Jour. Marine Research, v. 4, p. 189–197, 1941.
Gran, H. H.1912. Pelagic plant life. p. 307–386 in Murray and Hjort, Depths of the Ocean. London, Macmillan, 821 pp., 1912.
Gran, H. H.1927. The production of plankton in coastal waters off Bergen, March to April, 1922. Norwegian Fishery and Marine Investigations, v. 3, no. 8, 74 pp., 1927. Bergen.
Gran, H. H.1929.
“Investigation of the production of plankton outside the Romsdalsfjord 1926–1927”
. Conseil Perm. Internat. p. l'Explor. de la Mer, Rapports et Proc.-Verb., v. 56, 15 pp., 1929.
Gran, H. H.1930.
“The spring growth of the plankton at Möre in 1928–29 and at Lofoten in 1929 in relation to its limiting factors”
. Norske Vidensk. Akad. i Oslo, Skrifter, 1. Mat.-Naturv. Klasse, no. 5, p. 5–77, 1930.
Gran, H. H.1931.
“On the conditions for production of plankton in the sea”
. Conseil Perm. Internat. p. l'Explor. de la Mer, Rapp. et Proc.-Verb., v. 75, p. 37–46, 1931.
Gran, H. H.1933.
“Studies on the biology and chemistry of the Gulf of Maine. II. Distribution of phytoplankton in August, 1932”
. Biol. Bull.v. 64, p. 159–181, 1933.
Gran, H. H., and T. Braarud. 1935.
“A quantitative study of the phytoplankton in the Bay of Fundy and the Gulf of Maine (including observations on hydrography, chemistry, and turbidity)”
. Biol. Board of Canada, Jour., v. 1, p. 279–467, 1935.
Gran, H. H., and T. G. Thompson. 1930.
“The diatoms and the physical and chemical conditions of the sea water of the San Juan Archipelago”
. Puget Sound Biol. Sta., Pub., v. 7, p. 169–204, 1930.
Gross, F.1940.
“The osmotic relations of the plankton diatom Ditylum brightxswelli (West.)”
. Marine Biol. Assn. U. K., Jour., v. 24, p. 381–415, 1940.
Gunther, E. R.1936.
“A report on the oceanographical investigations in the Peru coastal current”
. Discovery Repts., v. 13, p. 107–276, 1936.
Harvey, H. W.1928. Biological chemistry and physics of sea water. Cambridge Univ. Press, 189 pp., 1928.
Harvey, H. W.1933.
“On the rate of diatom growth”
. Marine Biol. Assn. U. K., Jour., v. 19, p. 253–276, 1933.
Harvey, H. W.1937.
“The supply of iron to diatoms”
. Marine Biol. Assn. U. K., Jour., v. 22, p. 205–219, 1937.
Harvey, H. W.1939.
“Substances controlling the growth of a diatom”
. Marine Biol. Assn. U. K., Jour., v. 23, p. 499–520, 1939.
Harvey, H. W., L. H. N. Cooper, M. V. Lebour, and F. S. Russell. 1935.
“Plankton production and its control”
. Marine Biol. Assn. U. K., Jour., v. 20, p. 407–442, 1935.
Hensen, Victor. 1887. Über die Bestimmung des Planktons oder des im Meere treibenden Materials an Pflanzen und Thieren. Komm. zur Wissensch. Untersuch. d. Deutschen Meere in Kiel, Fünfter Bericht, XII–XVI Jahrg., p. 1–107, 1887.
Hentschel, E.1928.
“Die Grundzüge der Planktonverteilung im Südatlantischen Ozean”
. Internat. Rev. d. ges. Hydrobiol. u. Hydrogr., Bd. 21, p. 1–6, 1928.
Hentschel, E., and H. Wattenberg. 1930.
“Plankton und Phosphat in der Oberflächenschicht des Südatlantischen Ozeans”
. Ann. d. Hydrogr. u. Mar. Meteor., Bd. 58, p. 273–277, 1930.
Jenkin, Penelope M.1937.
“Oxygen production by the diatom Coscinodiscus excentricus Ehr. in relation to submarine illumination in the English Channel”
. Marine Biol. Assn. U. K., Jour., v. 22, p. 301–342, 1937.
Johnstone, J., A. Scott, and H. C. Chadwick. 1924. The marine plankton. Liverpool, Univ. Press, 194 pp., 1924.
Karsten, G.1905. Das Phytoplankton des Antarktischen Meers nach Material der Deutschen Tiefsee-Expedition 1898–1899. Wiss. Erg. d. Deutschen Tiefsee-Exped. auf dem Dampfer Valdivia 1898–1899, Bd. II, 2 Teil, 136 pp., 1905.
Klugh, A. B.1930. Studies on the photosynthesis of marine algae. No. 1. Photosynthetic rates of Entromorpha linza; Porphyra umbilicalis, and Delesseria sinuosa in red, green, and blue light. Contributions to Canadian Biol. and Fisheries, N.S., v. 6, p. 41–63, 1930.
Kofoid, C. A.1906.
“On the significance of the asymmetry in Triposolenia”
. Univ. Calif., Pub. Zool., v. 3, p. 127–133, 1906.
Kreps, E., and N. Verjbinskaya. 1930.
“Seasonal changes in the phosphate and nitrate content and in hydrogen ion concentration in the Barents Sea”
. Conseil Perm. Internat. p. l'Explor. de la Mer, Jour. du Conseil, v. 5, p. 329–346, 1930.
Krogh, A.1934. Conditions of life in the ocean. Ecological Monographs, v. 4, p. 421–429, 1934.
Lebour, Marie V.1922.
“The food of plankton organisms”
. Marine Biol. Assn. U. K., Jour., v. 12, p. 644–677, 1922.
Lohmann, Hans. 1908. Untersuchungen zur Feststellung des vollständigen Gehaltes des Meeres an Plankton. Komm. z. Wissensch. Untersuch. d. Deutschen Meere in Kiel und d. Biologischen Anstalt auf Helgoland, Wissensch. Meeresuntersuch., N.F., Abt. Kiel, Bd. 10, p. 131–370, 1908.
Lubimenko, V. N., and Z. Tikhouskaia. 1928.
“Recherches sur le photosynthèse et l'adaptation chromatique chez les algues marines”
. Sebastopol Biol. Sta., Trudy, v. 1, p. 153–190, 1928 (U.S.S.R. Akad. Nauk).
Marshall, Sheina. 1924.
“The food of Calanus finmarchicus during 1923”
. Marine Biol. Assn. U. K., Jour., v. 13, p. 473–479, 1924.
Marshall, S. M., and A. P. Orr. 1927.
“The relation of the plankton to some chemical and physical factors in the Clyde Sea area”
. Marine Biol. Assn. U. K., Jour., v. 14, p. 837–868, 1927.
Marshall, S. M., and A. P. Orr. 1928.
“The photosynthesis of diatom cultures in the sea”
. Marine Biol. Assn. U. K., Jour., v. 15, p. 321–360, 1928.
Moberg, Erik G.1928.
“Interrelation between diatoms, their chemical environment, and upwelling water in the sea off the coast of southern California”
. Nat. Acad. Sci., Proc., v. 14, p. 511–518, 1928.
Nathansohn, A.1906. Über die Bedeutung vertikaler Wasserbewegungen für die Produktion des Planktons im Meer. Kgl. Sächs. Gesellsch. der Wissensch., Abhandl. d. Math.-Phys. Klasse, Bd. 29, no. 5, 1906.
Ostenfeld, C. H.1909.
“Immigration of a plankton diatom into a quite new area within recent years: Biddulphia sinensis in the North Sea waters”
. Internat. Rev. d. ges. Hydrobiol. u. Hydrogr., Bd. II, p. 362–374, 1909.
Peters, N.1932.
“Die Bevölkerung des Südatlantischen Ozeans mit Ceratien. Deutsche Atlantische Exped. Meteor 1925–1927”
, Wiss. Erg., Bd. XII, 1 Teil, p. 1–69, 1932.
Pettersson, H., H. Höglund, and S. Landberg. 1934. Submarine daylight and the photosynthesis of phytoplankton. Oceanografiska Inst. Göteborg

Phifer, L. D.1933. Seasonal distribution and occurrence of planktonic diatoms at Friday Harbor, Washington. Univ. of Washington, Pub. in Oceanogr., v. 1, p. 39–81, 1933.
Schreiber, E.1927. Die Reinkultur von marinen Phytoplankton und deren Bedeutung fur der Produktionsfahigkeit des Meerswassers. Komm. z. Wissensch. Untersuch. der Deutschen Meere in Kiel und d. Biologischen Anstalt auf Helgoland, Wissensch. Meeresuntersuch., N. F., Abt. Helgoland, Bd. 16, p. 1–35, 1927.
Spoehr, H. A.1926. Photosynthesis. New York, Chemical Catalog Co., Inc., 393 pp., 1926.
Stanbury, F. A.1931.
“The effect of light of different intensities, reduced selectively and non-selectively, upon the rate of growth of Nitzschia closterium”
. Marine Biol. Assn. U. K., Jour., v. 17, p. 633–653, 1931.
Steemann Nielsen, E.1939.
“Über die vertikale Verbreitung der Phytoplanktonten im Meere”
. Internat. Revue d. ges. Hydrobiol. u. Hydrogr., Bd. 38, p. 421–440, 1939.
Sverdrup, H. U., and W. E. Allen. 1939.
“Distribution of diatoms in relation to the character of water masses off southern California in 1938”
. Jour. Marine Research, v. 2, p. 131–144, 1939.
Thompson, T. G., and R. W. Bremner. 1935.
“The occurrence of iron in waters of the northeast Pacific Ocean”
. Conseil Perm. Internat. p. l'Explor. de la Mer, Jour. du Conseil, v. 10, p. 39–47, 1935.
Thompson, T. G., and M. W. Johnson. 1930.
“The sea water at the Puget Sound Biological Station from September, 1928, to September, 1929”
. Puget Sound Biol. Sta., Pub., v. 7, p. 345–368, 1930.
ZoBell, Claude E.1935.
“The assimilation of ammonium nitrogen by Nitzschia closterium and other marine phytoplankton”
. Nat. Acad. Sci., Proc., v. 21, p. 517–522, 1935.