X. Observations and Collections at Sea [PDF]
OCEANOGRAPHIC VESSELS AND THEIR FACILITIES
Vessels
For purposes of oceanographic research, a very sturdy, seaworthy vessel capable of working under practically all weather conditions and of withstanding any storm is required. Vessels engaged in marine investigations can be broadly classified as either oceanic or coastal types, depending largely upon their size and cruising radius, but the two categories are not sharply defined, since large vessels may be used for near-shore investigations and relatively small vessels sometimes extend their operations far out to sea. In the following discussion, vessels and equipment used in coastal surveying and in the study of fisheries problems will not be described, although vessels engaged primarily in such work are sometimes employed in oceanographic investigations. Practically any vessel, small or large, can be used for certain types of investigations, but rarely is any single craft, unless specially designed, suitable for all kinds of oceanographic work. One of the chief requirements of oceanographic vessels operated by private or small organizations is economy of operation. This generally means a relatively small craft with low maintenance cost which can be handled by a small crew. Vessels owned or operated by national agencies, such as the Meteor (Germany), Discovery II (Great Britain), and Willebrord Snellius (Netherlands), are generally fairly large, but in most cases they serve a dual purpose. For example, the Meteor was used as a naval training ship and as a survey vessel, and the Snellius was especially built for surveying work in the Netherlands East Indies.
The following features are desirable in vessels that are to be used in oceanographic research:
Sturdiness and seaworthiness, large cruising radius, and accommodations for laboratory work and the storage of collections.
Low freeboard in order to make possible the handling of instruments near the sea surface and to reduce the wind drift when hove to at stations.
Sails to increase the cruising radius, to provide a safety factor in case of engine breakdown, and to improve the working conditions on board by reducing the roll and vibration when under way. Riding sails to steady the vessel when hove to at stations and to reduce the leeway by keeping the vessel headed into the wind.
Sufficient clear deck space for the installation of winches and for handling bulky equipment such as trawls and dredges.
Name of vessel | Nationality | Operated by | Type of vessel | Launched | Commissioned for oceanographic work | Overall length, feet | Tonnage | Officers and crew | Scientists | Reference |
---|---|---|---|---|---|---|---|---|---|---|
R.R.S. Discovery II | Great Britain | Discovery Committee of the Colonial Office | Steel steam vessel (trawler) (special) | 1929 | 1930 | 234 | 2100 (disp.) | 46 | 6 | Ardley and Mackintosh (1936) |
F. & V.S. Meteor | Germany | Hydrographic Department of the Navy | Steel steam vessel for survey and training (gunbost) | 1915 | 1924 | 233 | 1200 (disp.) | 114 | 10 | Spiess (1932a) |
H.M.S. Willebrord Snellius | Netherlands | Hydrographic Section, Department of Defense | Steel steam vessel for surveying (gunboat) | 1928 | 1929 | 204 | 1055 (disp.) | 84 | 6 | Pinke (1938) |
R.R.S. Dana (II)[a] | Denmark | Danis Commission for the Investigation of Sea | Steel steam vessel (trawler) | 1917 | 1921 | 138 | 360 (gross) | 14 | 8 | Schmidt (1929) |
Armauer Hansen | Norway | Geophysical Institute, Bergen | Wooden auxiliary ketch (special) | 1912 | 1913 | 76 | 57 (gros) | 5 | 6 | Helland-Hansen (1914) |
Carnegie[b] | U.S.A. | Department of Terrestrial Magnetism, Carnegie Institution of Washington | Wooden auxiliary brigantime (nonmagnetic) | 1909 | 1928[c] | 155 | 568 (disp.) | 17 | 8 | Bauer, Peters, Ault, Fleming (1917) |
Atlantis | U.S.A. | Woods Hole Ocean ographic Institution | Steel auxiliary ketch (special) | 1930 | 1931 | 142 | 460 (disp.) | 17 | 5 | Iselin (1933) |
E.W. Scripps | U.S.A. | Scripps Institution of Oceanography, University of California | Wooden auxiliary schooner (yacht) | 1924 | 1938 | 104 | 140 (disp.) | 7 | 6 | Moberg and Lyman (1942) |
Catalyst | U.S.A. | Oceanographic Laboratories, University of Washington | Wooden motor vessel (special) | 1932 | 1932 | 75 | 94 (gros) | 5 | 9 | Thompson (1936) |
In table 57 are listed certain representative vessels that have been extensively used in oceanographic investigations. Those owned by national agencies are large, over 200 feet long, and carry large crews, while, on the other hand, vessels owned and operated by institutions are generally between 100 and 150 feet long and carry crews of less than twenty. During the nineteenth century the practice of utilizing only large craft in oceanographic work made it impossible for private organizations to engage in independent and systematic investigations. However, Björn Helland-Hansen, of the Geophysical Institute in Norway, convinced that small vessels could be used effectively, had the Armauer Hansen built to conform to his ideas. This small vessel, only 76 feet long, has carried out both intensive and extensive work in the North Atlantic and has ably confirmed Helland-Hansen's thesis. Following his lead, other private institutions have purchased or built small vessels that can be economically operated.
Winches
Winches used in oceanographic investigations vary so widely in construction that it is impossible to describe any standard designs. The type and design of winches depend not only upon the character of the work contemplated but also upon the size of the vessel, the space available for the installation, the length of wire rope to be carried, and the power for operating the winch. Details of construction and installation of the winches on the vessels listed in table 57 may be found in the references cited.
Winches may be classified under three headings, depending almost entirely upon the strength of the wire rope they carry.
Sounding winches are relatively light. They carry single or multistrand wire of small diameter and are designed for sounding and for obtaining bottom samples with light gear. Sometimes they can be used for other types of oceanographic work. An electric powered deep-sea sounding winch is described by Parker (1932).
Hydrographic winches are moderately stout. They carry somewhat heavier wire than the sounding winches and are designed for handling water-sampling devices, thermometers, and plankton nets. Since the introduction of sonic sounding methods, winches especially designed for taking wire soundings are not so common, and hence hydrographic
Heavy winches are strongly built to carry the largest and strongest cables. They are used for dredging, trawling, anchoring in deep water, and for any other work requiring heavy equipment or the ability to withstand a great strain.
The construction of winches depends not only upon the size of wire rope handled, but also upon its length, since those carrying small amounts need not be so large or so strong as those that must handle several thousand meters of wire rope. For investigations in the open sea, winches should carry at least 5000 m of cable, and for studies in the deeps, more than 10,000 m may be required. Winches carrying only a few hundred meters of wire rope can be cheaply built and, if necessary, operated by hand. However, heavy winches and those carrying large amounts of wire are always power driven. Winches may be operated by steam, as on the Discovery II, by gasoline or diesel motors coupled directly to the winch, by the main engine through some suitable mechanism, or by electric motors. Because of its economy of operation and its flexibility, steam is in many ways the most desirable source of power for winches, but it is practical only on steam-driven vessels. Oceanographic winches are now most commonly operated by electric motors. It is essential that all winches, particularly those used in handling water-sampling devices and nets, have a considerable range in speed of lowering and hauling in. They must also be capable of being controlled quickly and accurately so that instruments can be lowered to a predetermined depth and raised to a convenient level above the water for examination or removal from the wire. The maximum rate of haul on the hydrographic winch should be about 200 m/min. If electric motors are used to operate winches, speed control is obtained by the use of rheostats. In certain installations, reduction in speed also reduces the horsepower of the motor, but such designs should be avoided, because heavy loads must be hauled in slowly.
Electric motors mounted on deck must be waterproof, and the winches themselves must be so constructed that they can be readily lubricated and protected from corrosion by salt water. Winches carrying large amounts of wire rope must have drums with extremely staunch flanges; otherwise, the packing of the wire may break the flanges away from the core when the winch is hauling in under tension.
Spreaders of some type are necessary to lay the wire smoothly and evenly on winch drums that carry large amounts of wire rope. If no provision is made for such spreaders, the wire may accumulate unevenly on the drum, causing the strands to break down and, what is more serious, causing the wire to slip down between the underlying coils in such a way that when payed out again it may be badly snarled. Spreaders may be
The hydrographic and sounding winches are customarily placed on deck near the rail, where they can be conveniently operated and where the wire rope will clear obstructions on deck and on the hull. The heavy winch, which is more bulky, must be installed in such a way that it can be strongly supported, can withstand heavy loads, and will not affect the stability of the vessel. On smaller craft it is commonly installed amidships below deck or, sometimes, half sunk below the deck level. The axis of rotation of the heavy winch is generally athwartships.
Wire Ropes and Accessory Fittings
Wire ropes used in oceanographic work must have the following properties:
They must be of strong material so that rope of relatively small diameter can be used, thus reducing the bulk of the winch.
They must be flexible and not liable to kink or unravel.
They must be made of a metal that is resistant to corrosion, and thus long-lived, and free from materials that will contaminate water samples and plankton catches.
Such requirements are best answered by multistrand ropes of stainless steel, but this alloy is expensive, and in practice tinned or galvanized steel ropes are satisfactory. Phosphor-bronze and aluminum-bronze ropes are also used, as they are noncorroding, but they are only about one half as strong as steel and their life is limited because they crystallize with use and lose their strength. Many wire ropes have hemp cores, but these are not so satisfactory as ropes with wire cores, because the hemp may shrink and break when submerged, and it is also liable to rot unless specially treated. High-grade manila rope is about one tenth as strong as steel rope of comparable diameter.
The strength of steel depends upon its composition and treatment and varies from about 50,000 to 400,000 lb/in2. Steels employed in wire ropes are usually of relatively high tensile strength, the tensile strength of the rope increasing with decreasing diameter of the individual wires. The greater strength of a rope made up of smaller but more numerous wires is offset, however, by the greater surface offered for corrosion and by the greater likelihood of the individual wires breaking after a certain amount of wear.
Single-strand wire of the type known as piano or music wire is used for deep-sea sounding and for running taut-wire traverses (p. 342). This wire is of extremely high tensile strength, but is stiff and liable to break if kinked. If no bottom sample is required when sounding at great depths and in taut-wire traverses, the wire is usually cut away, as it is not worth the time required to reel it in again. Piano wire of
The wire ropes used on the hydrographic and heavy winches are generally of either the 7 × 7 or the 7 × 19 types. A 7 × 7 wire rope consists of six strands, each composed of seven individual wires that are wound around a central core strand which itself contains seven wires. A 7 × 19 wire rope is similarly constructed, but each of the seven strands contains nineteen individual wires. The 7 × 19 ropes are slightly heavier than the 7 × 7 type, and the ropes of smaller diameter are considerably stronger, but the small size of the individual wires is a disadvantage. Ropes of the 7 × 7 type with diameters between about one eighth inch and one quarter inch are sufficiently flexible for oceanographic use, but, to obtain the desired flexibility in larger ropes, it is necessary to employ 7 × 19 rope on the heavy winch. In table 58 are given the characteristics of 7 × 7 galvanized steel ropes of the type known as aircraft cord.
For most purposes it is considered that the working load of wire rope should not be more than one fifth of the breaking strength—that is, a safety factor of five. In marine investigations it is sometimes impossible to maintain such a high factor of safety, but, if the anticipated strain is known, the diameter of the wire should be such that the maximum load is never more than half the breaking strength. There is not only the danger of losing valuable equipment, but also the hazard to those on deck if the wire should break near the water surface. Steel has a greater elasticity than bronze, and the safety factor must be somewhat greater when bronze ropes are employed.
When great lengths of wire rope are paid out, the weight of the rope in the water may approach the breaking strength and exceed the safety factor of five, even when there is no gear suspended from the rope. Ropes of the type listed in table 58 exceed the safety factor of five when more than 4000 m of wire are suspended in the water. When taking water samples and temperatures or other observations with light gear where there are no sudden strains upon the rope, the safety factor may be reduced and the work extended to great depths. However, in trawling, dredging, and taking cores of the bottom sediments, and when anchoring in deep water, the equipment must be such that it can withstand a heavy working load in addition to the weight of the wire rope. The increased working strength necessary for observations in deep water is gained by using tapered wire ropes, which are of the smallest diameter
Diameter | Weight in air per 100 m | Breaking strength | |||
---|---|---|---|---|---|
Millimeters | Inch | Kilograms | Pounds | Kilograms | Pounds |
Data through Courtesy of John A. RoebIing's Sons Co., Trenton, New Jersey. | |||||
3.18 | 1/8 | 3.6 | 8.0 | 610 | 1,350 |
3.97 | 5/32 | 6.9 | 15.3 | 1,180 | 2,600 |
4.76 | 3/16 | 8.6 | 19.0 | 1,450 | 3,200 |
5.56 | 7/32 | 12.3 | 27.2 | 2,090 | 4,600 |
6.35 | 1/4 | 15.6 | 34.4 | 2,630 | 5,800 |
7.94 | 5/16 | 24.9 | 54.8 | 4,200 | 9,200 |
9.52 | 3/8 | 34.2 | 75.5 | 5,900 | 13,100 |
11.10 | 7/16 | 45.8 | 101.0 | 7,400 | 16,400 |
12.70 | 1/2 | 62.6 | 138.0 | 10,200 | 22,500 |
Many preparations are on the market to be used for the preservation of wire ropes. Whether or not any of these are suitable depends upon the manner in which the wire rope is to be used, as those which flake off will contaminate plankton samples and other collections. Relatively frequent application of used crankcase oil is a satisfactory method for preserving steel ropes. The oil is applied before the rope is first placed in the water, and thereafter at intervals, particularly when the rope will not be in service for a long period.
The wire rope used on the hydrographic winch must be smooth and free from kinks or stray broken wires that will prevent the passage of messengers or weights. Where a break or other damage necessitates a join, wire rope may be repaired with a long splice, which does not materially increase the diameter of the wire or decrease the breaking strength. Kinks that form in the wire should never be pulled out, but should be eliminated by untwisting the wire, straightening the strands, and realigning them.
Sheaves are aIways necessary for leading the wire rope outboard. They should be free-running and of such diameter that the wire passing over them will not be cramped or strained. Unless the circumstances
An essential part of the equipment for each winch is a meter wheel (fig. 83) for measuring the amount of rope payed out. A meter wheel is a sheave with a wheel of appropriate circumference fitted with a device that records the number of revolutions. The device is so designed that the difference between two readings of the dials gives directly in meters, fathoms, or feet the amount of wire that is run out or hauled in. For soundings and for obtaining temperatures and water samples the meter wheel must be carefully constructed and checked from time to time for wear. The effective diameter of the wheel is its own diameter plus the diameter of the wire rope. The meter wheel may be mounted on the boom or davit that leads the wire outboard, or it may be built into the spreader on the winch. The latter arrangement is a convenient one because the winch operator can then see the recorder at all times. If the meter wheel itself is in such a location that the dial is not readily seen, recorders operated by a flexible speedometer cord may be mounted in a more convenient place. To avoid slippage of the wire rope over the meter wheel, the angle of contact should be at least 90 degrees.
The wire rope is led outboard from the winch through sheaves and finally from a boom or davit extending out over the side of the vessel on the windward side. The lead from the heavy winch must be approximately amidships because, when dredging, the vessel must be maneuvered under sail or power, which is possible only if the wire rope is suspended from a sheave near the middle of the vessel. To avoid striking the side of the vessel the outboard leads should be arranged so that the wire, when hanging vertical, is several feet away from the hull, and to facilitate the handling of gear it is usually necessary to have a working platform extending out from the hull and large enough to accommodate two men. If the wire rope on the heavy winch is used for deep-sea anchoring,
Sudden strains on the wire rope that may be caused by the rolling of the vessel or by fouling equipment on the bottom are a hazard to both the apparatus and the wire. These strains may be equalized somewhat by the use of accumulators, which are usually coil springs mounted with one end secure and the other end attached to the sheave through which the wire rope passes. Accumulators can usually be calibrated so that the extension or compression of the spring may be used to measure the strain on the wire rope. Some types are secured to the outer end of the boom or davit with the meter wheel or a plain sheave attached to the free end, or they may be an integral part of the davit or winch. The strain on the accumulator may be used in deep soundings to determine when the weight strikes the bottom, and in dredging and trawling it should be watched so that sudden strains may be eased by slacking off on the winch. Special devices known as dynamometers may be used to measure the strain on the wire rope.
Shipboard Laboratories
The location of laboratories on shipboard, the amount of space devoted to them, and the facilities installed depend upon the size and nature of the vessel and the types of investigations to be made. The laboratories may be classified as deck laboratories and analytical laboratories. The deck laboratory opens on the deck and is used for storing certain oceanographic equipment. There the reversing thermometers are read, water samples are drawn from the sampling device, and certain preliminary steps are taken in the preservation or preparation of water and plankton samples. The deck laboratory should contain racks in which the water-sampling bottles may be placed as soon as they are removed from the wire rope. These racks should be so arranged that the temperatures can be read and the water samples can be taken out without removing the bottles. Space should be provided for the glass bottles in which the water samples are transported, for certain chemical reagents, and for the solutions required for the preservation of biological material. A bench where records and labels can be prepared is also a great convenience. A large sink with running fresh and salt water is very useful, and the deck should be watertight and provided with drains because water is spilled in filling the glass bottles. Deck laboratories are a great asset on oceanographic vessels, particularly in bad weather and at night, as much more satisfactory work can be done under shelter where there is good illumination.
The analytical laboratories are usually located below decks where there is the most space and where the motion of the vessel is at a minimum.
Vibration transmitted through the vessel from the engines and motors is often more troublesome in the laboratory than the roll or pitch of the vessel, and consequently the engines should be mounted, when practicable, on flexible springs or on cork or rubber. The equipment used in the laboratories will generally be identical with that used on shore, but the benches, storage space, and methods of securing apparatus to the benches must be adapted to work at sea under any conditions. Work benches are usually of such a height that the worker can be seated on a stool or seat that is fixed in place and so arranged that he can brace himself with his legs, thus leaving his hands free. In some cases provisions are made for a bench mounted on gimbals, but the advantages of a relatively level surface are often outweighed by its unsteadiness. All apparatus must have suitable storage compartments in which there is no danger of the apparatus falling out or smashing together in a high sea. Burettes and other instruments, while in use, must be secured to the bench or to permanent burette stands.
The analytical laboratory should be provided with running fresh water and a source of distilled water. The latter may be carried in large bottles or, preferably, in specially installed tin-lined tanks. On a long cruise it may be necessary to provide distilling apparatus. When living organisms are to be investigated, a source of cooled sea water or a cold box is necessary. A cold box is also desirable for preserving water or sediment samples for bacteriological examination.
Laboratory work on shipboard is usually kept to a minimum because of the undesirable working conditions that arise from the cramped space, the motion of the vessel, vibration, and the time required for merely collecting the samples. However, there are certain chemical tests that must be made immediately after the samples are collected, and generally these must be made on board. The methods of analysis are referred to in chapter VI. On longer cruises it may be necessary to do more of the work on board, but in such cases the analyses may be done when the weather conditions are favorable or when the vessel is in port or at anchor. Biological work on board the vessel is limited in character, since most specimens can be preserved for later examination ashore and because vibration and motion of the vessel make the use of microscopes virtually impossible.
Samples of water, organisms, or sediments that are to be examined ashore are usually not stored in the laboratory but must be kept in a place not subject to wide ranges in temperature or to extreme temperatures. High temperatures lead to the disintegration of the rubber washers used on most bottles and thus permit evaporation, which will ruin the specimens. Fluctuating temperatures may loosen the stoppers and lead to evaporation, or may even break the bottles. Freezing temperatures must also be avoided, owing to the danger of breakage.
OBSERVATIONS AND COLLECTIONS
Positions at Sea
The geographical location at which an observation is made must be known. The greater frequency with which observations are now taken makes it necessary to know the locations of sampling very accurately, and hence special methods of determining positions at sea have been developed. Accurate knowledge of locations is particularly necessary in surveying, where the introduction of sonic sounding methods has made it possible to take large numbers of soundings that must be precisely plotted in order to bring out the true configuration of the bottom. The specialized techniques developed by such organizations as the U. S. Coast and Geodetic Survey have not yet been used for general oceanographic work, but the methods may be adopted for the study of special problems.
When in sight of land where recognizable features are accurately located, the position of the vessel may be determined by means of horizontal angles and bearings on shore features. Out of sight of land the position can be determined by astronomic sights or by radio direction-finder bearings. Between positions established in these ways the location at any time is obtained by dead reckoning—namely, from the course steered and the distance run. Such methods are adequate for most oceanographic work, but, where greater accuracy is required, as in offshore surveying, positions found in this way are not commensurate with the accuracy and frequency of soundings. In certain cases an anchored vessel or buoy whose position can be exactly established by repeated astronomic observations is used as a point of reference. Since 1923 the U. S. Coast and Geodetic Survey has experimented with sonic methods of locating positions and has developed them to a high degree of accuracy. In radioacoustic ranging (usually designated as R.A.R.), the surveying vessel drops a depth bomb that is fired by fuse or electricity. The sound of the explosion is picked up by a hydrophone on the vessel and recorded on a chronograph. The impulse of the explosion, which travels in all directions, is picked up by hydrophones attached to shore stations, anchored vessels, or buoys whose positions are accurately known. The hydrophones are connected to radio transmitters, and the sound impulse received at each hydrophone is transmitted by radio to the surveying vessel, where the times of reception are automatically recorded on the chronograph. Since the time required for the transmission and reception of the radio signal is infinitesimal, the period between the bomb explosion and the return of the signal from each hydrophone is that required for the sound impulse to travel through the water from the point of the bomb explosion to the hydrophone. The velocity of sound in water can be computed from the known distribution of temperature and salinity
Taut-wire traverses are also used to determine accurately the distances between anchored buoys when detailed surveys are made out of sight of land. The distances are measured by paying out steel piano wire under controlled tension over an accurate meter wheel from drums which carry over 140 miles of wire. When the distance between a row of anchored buoys 15 or 20 miles long has been measured, the wire is cut and abandoned. With a combination of the methods outlined above, extremely accurate surveys can be made out of sight of land where depths are not too great to make it impossible to anchor the buoys satisfactorily. The methods employed by the Coast and Geodetic Survey have been described by Rudé (1938) and by Veatch and Smith (1939).
Sonic Soundings
Sonic-sounding equipment consists of three essential parts: (1) a source that will emit a sound impulse, (2) an instrument for detecting or recording the outgoing and the returning signals, and (3) a means of measuring the time required for the sound to travel to the sea bottom and for the echo to return to the ship. Sound sources are of two general types: those that emit sound of audible frequency which is nondirectional in water, or those that emit high-frequency vibrations which are nonaudible and are classed as ultrasonic. The audible type is satisfactory for general use, but for sounding in shoal water or over a bottom that is very irregular, directional ultrasonic equipment must be used. Audibletype transmitters usually consist of a diaphragm that is vibrated by an electromagnet, although other devices are employed. The ultra- or supersonic transmitter depends upon the piezoelectric property of quartz crystals which, when subjected to a high voltage, vibrate at high frequency, and, as the process is reversible, the returning echo stimulates a current through the circuit, so that the same device is used as a transmitter and as a sound detector. In the audible-type sonic sounder the outgoing signal and the returning echo are picked up by a submerged microphone called a hydrophone.
Various devices are used to determine the time required for the sound impulse to travel to the bottom and return to the hydrophone. For sounding in deep water the simplest method is to measure the
Wire Soundings
Since the introduction of sonic methods, relatively few wire soundings are taken for the sole purpose of measuring depths. Checks must be made from time to time to see that the sonic equipment is operating successfully, but in most cases wire soundings are now made for the express purpose of obtaining samples of the sea-bottom sediments. The weight of equipment used for collecting bottom samples has increased, and, as a consequence, the piano-wire sounding machines are no longer
An unprotected reversing thermometer for measuring pressure, used in conjunction with a protected reversing thermometer and attached to the sounding wire some 50 m above the weight, is sometimes used as a check on the depth (p. 351).
Bottom-Sampling Devices
Devices used for collecting specimens of the sea-bottom sediments depend upon the character of the deposit, the depth of water, and the strength of the wire rope available. Certain apparatus that is suitable for work in soft, cohesive sediments cannot be used where the bottom material is coarse-grained or where it is rocky. Similarly, other types that can be operated in shallow depths are too heavy for general use in deep water. Methods have been devised for sampling the superficial layers of the sediments, but since the Meteor Expedition, 1925–1927, greater emphasis has been placed upon obtaining core samples. Instruments are now in use that will take cores several meters long, and much thought is being given to the development of instruments that will take even longer samples. Unlike the equipment used in most of the other fields of oceanography, the devices for taking samples of the marine sediments are not standardized. Every investigator uses his own type of bottom sampler, but all samplers are based on certain basic designs. Hough (1939) has listed and described the various types and gives an exhaustive bibliography.
Bottom samplers used for oceanographic work fall into three general categories; dredges (drag buckets), snappers, and coring tubes. Dredges patterned after the naturalist dredge (fig. 89), but constructed of stronger material and with a chain-mesh bag, are used for procuring samples of rock where the sea bottom is covered with rock fragments or where there are outcrops of solid rock. Smaller cylindrical dredges with solid bottoms
Coring devices (fig. 85) are essentially long tubes that are driven into the sediment, either by their own momentum or by the discharge of an explosive. The latter principle is used in the coring instrument developed by Piggot (1936). A momentum-type coring instrument weighing about 600 lb with the weights attached will take cores up to about 5 m in length in soft sediments at depths as great as about 2000 m (Emery and Dietz, 1941). The Piggot coring tube has been used to take cores about 3 m long at depths greater than 4000 m. In coarse-grained deposits the coring tubes are not capable of penetrating more than about 0.5 m.
The momentum-type core sampler is allowed to run out freely when it approaches the bottom. The depth of penetration is determined by the weight of the instrument, the character of the sediment, the diameter of the tube, and the type of cutting nose. The factor that determines the size and type of coring tube which can be used on any wire rope is not the weight of the instrument alone, because the greatest strain is developed when the coring tube is pulled out of the sediment, and this may be several times the weight of the instrument. The Emery-Dietz sampler is constructed of galvanized iron pipe of 2- or 2.5-inch diameter. It is connected through a reducing coupling to a smaller pipe on which the weights are mounted. The reducing coupling is perforated to permit the passage of water, and is sometimes fitted with a ball valve. The cutting nose is sharp, with a slightly smaller internal diameter than the tube itself so as to reduce the internal wall friction and to hold in the inner liner, which further reduces the wall friction and facilitates the removal of the core samples. Inner liners are sheets of metal or celluloid rolled up and inserted in the pipe, or they are glass or metal pipes that are cut open after the sample is taken. In some coring devices a “core catcher” is fitted to the nose of the coring tube to prevent the core from sliding out of the tube after the instrument is raised from the bottom. The depth of penetration of coring tubes is generally considerably greater than the length of the sample obtained. The amount of “compaction” varies between about 25 and 50 per cent, depending upon the characteristics of the coring tube and the type of sediment. The nature of the compaction and the effects that it may have upon the stratification of the sample have been investigated by Emery and Dietz (1941). Smaller instruments based on an original design by Ekman and developed by Trask (Hough, 1939), but of the same general type described above, can be used on fairly light gear and will take samples between 0.5 and 1.0 m in length.
The care and treatment of bottom samples depend to a large extent upon the nature of the examinations that are to be made later (chapter XX). For most purposes it is sufficient to place the specimens in mason jars fitted with rubber washers. No preservative or additional water is necessary. Labels should be placed on the outside of the bottle, since mechanical abrasion and the activities of microorganisms may destroy or render illegible any paper in contact with the sediment sample. Core samples may be cut in sections and carefully placed in bottles, or the entire specimen may be kept in the inner liner.
Temperature Measurements
Three types of temperature-measuring devices are used in oceanographic work. Accurate thermometers of the standard type are employed for measuring the surface temperature when a sample of the surface water is taken with a bucket and for determining the subsurface temperatures when the water sample is taken with a thermally insulated sampling bottle (p. 354). The thermometers used for measuring temperatures at subsurface levels are of the reversing type and are generally mounted upon water-sampling bottles so that temperatures and the water sample for salinity or other chemical and physical tests are obtained at the same level. The third type are temperature-measuring instruments that give a continuous record, such as thermographs, which are employed at shore stations and on board vessels to record the temperature at some fixed level at ot near the sea surface. Many devices have been invented for
The centigrade scale is the standard for the scientific investigation of the sea. A high degree of accuracy is necessary in temperature measurements because of the relatively large effects that temperature has upon the density and other physical properties and because of the extremely small variations in temperature found at great depths. Subsurface temperatures must be accurate to within less than 0.05°C, and under certain circumstances to within 0.01°. Such accuracy can be obtained only with well-made thermometers that have been carefully calibrated and rechecked from time to time. Because of the greater variability of conditions in the surface layers the standards of accuracy there need not be quite so high.
Conventional-type thermometers for surface temperatures or for use with an insulated bottle must have an open scale that is easy to read, with divisions for every tenth of a degree. The scale should preferably be etched upon the glass of the capillary. The thermometer should be of small thermal capacity in order to attain equilibrium rapidly; it should also be checked for calibration errors at a number of points on the scale by comparison with a thermometer of known accuracy, and it should be read
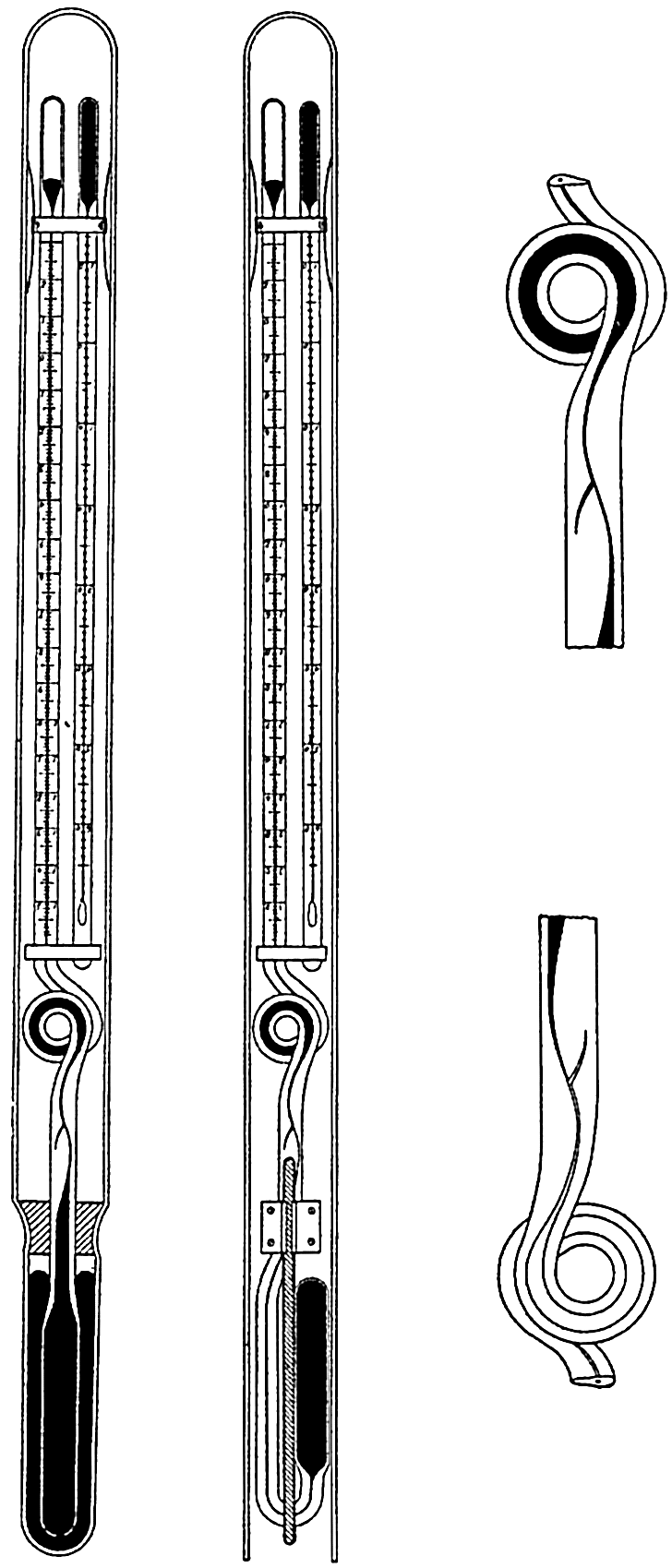
Protected and unprotected reversing thermometers in set position, that is, before reversal. To the right is shown the constricted part of the capillary in set and reversed positions.
[Full Size]
Protected Reversing Thermometers. Reversing thermometers (fig. 86) are usually mounted upon the water-sampling bottles (fig. 87), but they may be mounted in reversing frames and used independently. Reversing thermometers were first introduced by Negretti and Zambra (London) in 1874, and since that time have been improved, so that well made instruments are now accurate to within 0.01°C. On the Challenger Expedition, 1873–1876, the subsurface temperatures were measured by means of minimum thermometers, which were the most satisfactory instrument available at that time.
A reversing thermometer is essentially a double-ended thermometer. It is sent down to the required depth in the set position, and in this position it consists of a large reservoir of mercury connected by means of a fine capillary to a smaller bulb at the upper end. Just above the large reservoir the capillary is constricted and branched, with a small arm, and above this the thermometer tube is bent in a loop, from which it continues straight and terminates in the smaller bulb. The thermometer is so constructed that in the set position mercury fills the reservoir, the capillary, and part of the bulb. The amount of mercury above the constriction depends upon the temperature, and, when the thermometer is reversed, by turning through 180 degrees, the mercury column breaks at the point of constriction and runs down, filling the bulb and part of the graduated capillary, and thus indicating the temperature at reversal. The loop in the capillary, which is generally of enlarged diameter, is designed to trap any mercury that is forced past the constriction if the temperature is raised after the thermometer has been reversed. In order to correct the reading for the changes resulting from differences between the temperature at reversal and the surrounding temperature at the time of reading, a small standard-type thermometer, known as the auxiliary thermometer, is mounted alongside the reversing thermometer. The reversing thermometer and the auxiliary thermometer are enclosed in a heavy glass tube that is partially evacuated except for the portion surrounding the reservoir of the reversing thermometer, and this part is filled with mercury to serve as a thermal conductor between the surroundings and the reservoir. Besides protecting
Readings obtained by reversing thermometers must be corrected for the changes due to differences between the temperature at reversal and the temperature at which the thermometer is read, and for calibration errors. An equation developed by Schumacher is commonly used for correction:
ΔT is the correction to be added algebraically to the uncorrected reading of the reversing thermometer, T′, t is the temperature at which the instrument is read, V0 is the volume of the small bulb and of the capillary up to the 0°C graduation expressed in terms of degree units of the capillary, and K is a constant depending upon the relative thermal expansion of mercury and the type of glass used in the thermometer. For most reversing thermometers, K has the value 6100. The term I is the calibration correction, which depends upon the value of T′. Where there are large numbers of observations to be corrected, it is convenient to prepare graphs or tables for each thermometer from which the value of ΔT can be obtained for any values of T′ and t and in which the calibration correction is included.Reversing thermometers are usually used in pairs, most commonly attached to the water-sampling bottles, but they may be mounted in special reversing frames that are either operated by messenger or that have a propeller release. The frames holding the thermometers are brass tubes which have been cut away so that the scale is visible and which are perforated around the reservoir. The ends of the tubes are fitted with coil springs or packed with sponge rubber so that the thermometers are firmly held but are not subject to strains.
Unprotected Reversing Thermometers. Reversing thermometers identical in design with those previously described but having an open protective tube are employed to determine the depths of sampling (fig. 86). Because of the difference in the compressibility of glass and mercury, thermometers subjected to pressure give a fictitious “temperature” reading that is dependent upon the temperature and the pressure. This characteristic is utilized for determining the depth of reversal. Instruments used for this purpose are so designed that the apparent temperature increase due to the hydrostatic pressure is about 0.01°C/m. An unprotected thermometer is always paired with a protected thermometer, by means of which the temperature in situ, Tw, is determined. When Tw, has been obtained by correcting the readings of the protected thermometer, the correction to be added to the reading of the unprotected thermometer, T′u, can be obtained from the equation
Special Devices. Temperatures measured by the methods mentioned above yield observations at discrete points in space and time. Subsurface observations with reversing thermometers are time consuming and the instruments and equipment are expensive. Many devices have been suggested for obtaining continuous observations at selected levels or as a function of depth. Thermographs are commonly used at shore stations and on vessels to obtain a continuous records at or near the sea surface. The thermometer bulb, usually containing mercury, is mounted on the ship's hull or in one of the intake pipes and connected to the recording mechanism by a fine capillary. The recording mechanism traces the temperature on a paper-covered revolving drum. Records of temperatures obtained by a thermograph should be checked at frequent intervals against temperatures obtained in some other way.
Various types of electrical-resistance thermometers have been designed to be lowered into the water and to give a continuous reading, but have not proved satisfactory. Spilhaus (1938, (1940) has developed an
Water-Sampling Devices
The types of water-sampling devices that will be described are those that are intended for taking samples at subsurface levels for physical and chemical studies. Samples for the enumeration of phytoplankton and for bacteriological examination may be obtained with these instruments, but for such purposes specially designed samplers are more commonly used. A water sampler for collecting at subsurface levels is so designed that it can be closed watertight at any desired depth, and thus the enclosed sample is not contaminated by water at higher levels or lost by leakage after the bottle is brought on board. Because of the great pressures encountered in deep water the sampling bottles are sent down open and then closed at the required depths by means of messengers or propeller releases. To expedite work at sea, water-sampling devices are used in series—that is, with more than one bottle on the wire rope so that samples can be taken at a number of depths on the same cast. As it is essential that temperatures and water samples be taken at the same depths, the water-sampling bottles are fitted with frames in which one or more reversing thermometers are placed. An exception to this is the insulated Pettersson-Nansen bottle, which can be used for the upper few hundred meters (p. 354). Water-sampling devices must be constructed of noncorrosive materials that will reduce contamination of the water samples to a minimum. The bottles are usually made of brass, plated inside with tin or silver or coated with a special lacquer. For removing the water sample they are fitted with a drain cock and an air vent. To be clearly visible when hauling in, the bottles should be painted white. Many types of sampling bottles have been invented, but the rigorous working conditions and the desirable features that have been listed above have reduced the types in general use to only a few of simple but rugged design.
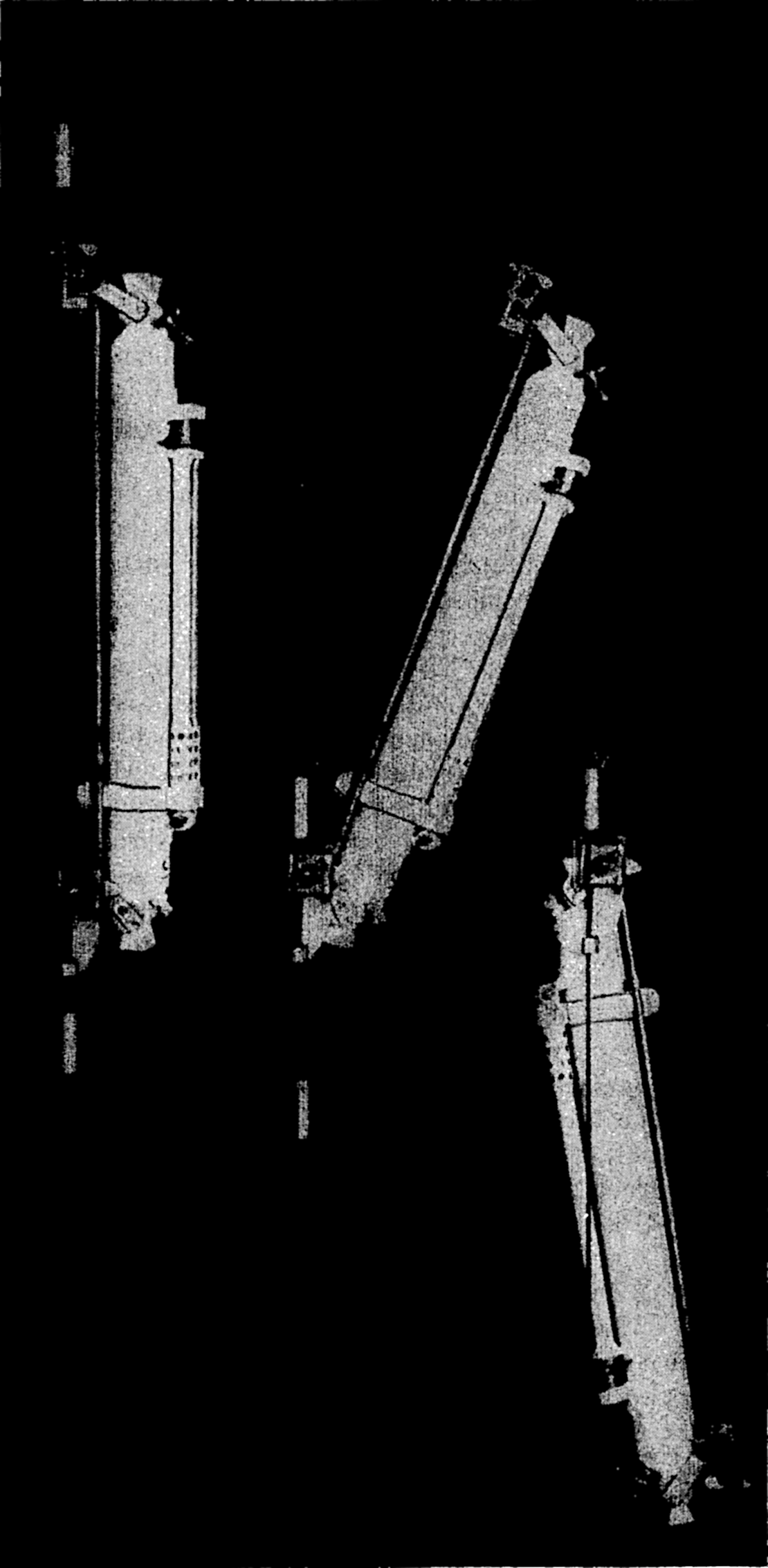
The Nansen reversing water bottle. Left: Before reversing; first messenger approaches releasing mechanism. Middle: Bottle reversing; first messenger has released the second. Right: In reversed position.
[Full Size]
Messengers are essential for the operation of many types of oceanographic equipment. Although their size and shape will vary for different types of apparatus, they are essentially weights which are drilled out so that they will slide down the wire rope. In order to remove or attach them they are either hinged or slotted. The speed of travel depends upon the shape and weight of the messenger and upon the wire angle (the angle the wire rope makes with the vertical). With no wire angle the messengers used with the Nansen bottles travel approximately 200 m per minute.
Water-sampling devices are of two general types, depending upon the method of closing, which may be accomplished by means of plug valves or by plates seated in rubber. The Nansen bottle, an example of the first type, is the one most widely used in oceanographic research. The Ekman bottle is of the second type.
The Nansen bottle (fig. 87) is a reversing bottle fitted with two plug valves and holding about 1200 ml. The two valves, one on each end of the brass cylinder, are operated synchronously by means of a connecting rod fastened to the clamp that secures the bottle to the wire rope. When the bottle is lowered, this clamp is at the lower end, and the valves are in the open position so that the water can pass through the bottle. The bottle is held in this position by the release mechanism, which passes around the wire rope, but, when a messenger sent down the rope strikes the release, the bottle falls over and turns through 180 degrees, shuts the valves, which are then held closed by a locking device, and reverses the attached thermometers. After reversing the bottle, the messenger releases another messenger that was attached to the wire clamp before lowering. This second messenger closes the next lower bottle, releasing a third messenger, and so on.
The Ekman bottle, which can also be operated in series, consists of a cylindrical tube and top and bottom plates fitted with rubber gaskets. The moving parts are suspended in a frame attached to the wire rope, and, when the instrument is lowered, the water can pass freely through the cylinder. When struck by a messenger the catch is released and the cylinder turns through 180 degrees, thereby pressing the end plates securely against the cylinder and enclosing the water sample. Reversing thermometers are mounted on the cylinder.
Thermally insulated bottles such as that of Pettersson-Nansen (for illustration, see Murray and Hjort, 1912) consist of several rigidly fixed concentric cylinders each of which is fitted with end plates. When these plates are shut, a series of water samples are isolated one within the other. The outermost cylinder and end plates are constructed of brass and ebonite, the inner ones of brass and celluloid, the cylinders
On the Meteor Expedition a glass-lined sampling bottle with a capacity of 4 l was used to collect large samples with a minimum of contamination. This bottle was attached to the end of the wire rope, the closing mechanism being similar to that used for the insulated bottle. A special mounting that reversed when the bottle was closed was provided for thermometers (Wüst, 1932).
In connection with wire sounding and bottom sampling, it is often desirable to obtain the temperature and the water samples close to the bottom and, as an added check, to obtain the depth by means of an unprotected thermometer. In order to avoid waiting for a messenger to travel all the way to the bottom, possibly one half hour or more, special sampling devices activated by propeller releases are used (Soule, 1932; Parker, 1932). These are usually reversing bottles in which the release pin is attached to a propeller. When the apparatus is being lowered, the propeller holds the pin in place, but, as soon as hauling in is commenced, the propeller rotates and withdraws the pin.
Spilhaus (1940) has devised a multiple sampler consisting of six small valve-closing bottles that can be closed individually at predetermined depths by releases which are activated by the hydrostatic pressure. The instrument was designed to be used in conjunction with the bathythermograph (p. 352) from a vessel while under way.
The general procedure for taking water samples and temperatures by means of Nansen bottles or other serial sampling devices is as follows. In order to keep the wire rope taut and to reduce the wire angle, a stray weight is attached to the end of the wire rope, usually of 50 to 100 lb, depending upon the size of the rope, the depths at which observations are to be taken, and the general working conditions. A certain amount of the wire rope (25 to 50 m) is then payed out so that the weight will not strike the ship when the bottom bottle is being attached or detached, and also to reduce the possibility of damaging the bottle if the weight strikes bottom.
The depth at which samples are to be collected should be planned before the cast is begun. The first bottle, adjusted to the set position, is then attached to the wire rope, the thermometers are checked, and the meter wheel is set at zero. When the wire has been lowered the required
Just before the messenger is released the wire angle should be estimated or measured. Knowledge of the wire angle is useful when determining the depth of sampling, as will be shown later.
Certain accessories are necessary when handling water-sampling bottles. As a safety measure a light line with a harness snap should be attached to the rail. This line is snapped on the bottle before it is handed to the operator on the working platform and is not removed until the bottle is firmly clamped on the wire rope. It is also attached when removing the bottle. To hold the wire rope steady and close to the platform a short line with a large hook should be attached to the platform. The hook is placed on the wire rope when instruments are being attached to it or removed from it. When the vessel is drifting with wind or surface currents, the wire will not hang vertically and will sometimes trail so far away that the wire angle may be as much as 50 or 60 degrees. Under these circumstances the wire rope must be pulled in by means of a boat hook or block and tackle in order to attach the hook.
Because of the larger vertical gradients in the distribution of properties in the upper layers, observations are taken at relatively close intervals near the surface and at increasingly larger intervals at greater depths. The International Association of Physical Oceanography,
TREATMENT AND ANALYSIS OF SERIAL OBSERVATIONS
After a number of temperature observations, salinity determinations, or other analyses have been made, it is necessary to eliminate those values that are in error and to put the data into a convenient form to make them readily comparable with other material of a similar character. The methods by which observations obtained with reversing thermometers are corrected to yield the temperatures in situ and by which the depths of sampling can be computed from the readings of unprotected thermometers have been described on preceding pages. The determination of salinity by chlorinity titration and other methods is discussed in chapter III. In addition, numerous chemical tests and analyses may be made on the water samples by methods listed in chapter VI. After the temperature in situ and the thermometric depths have been calculated, the observational data are generally listed on a summary sheet which shows the following information about each water sample: wire depth of each sampling instrument (the amount of wire rope between the surface and the sampling device), wire angle for each cast, temperature in situ (the corrected protected thermometer reading, or, if two thermometers were attached to the bottle, the average), depth of sampling as computed from the unprotected thermometer (these are usually not attached to each bottle), chlorinity, salinity, and other analyses that have been made.
The next step in the analysis of serial oceanographic observations is to establish the depths of sampling. A considerable amount of personal judgment enters, and this can be improved only by general experience, knowledge of the area in which observations were made, and familiarity with the behavior of individual instruments. The general procedure that must be followed is somewhat as follows: (1) if there was any error in the meter wheel, the necessary corrections must be applied to the meter-wheel readings; (2) if the wire angle was 5 degrees or less, the depths of sampling can be taken as equal to the wire depths as
After the accepted depths have been decided upon, the next step in the analysis of the observations is to plot vertical distribution curves. In one diagram, one of the observed properties may be plotted as a function of depth at a number of stations, or all of the observed properties at a single station may be plotted. From such curves it is often possible to detect those samples that are incorrect because of faulty functioning of the thermometers or because the water-sampling device closed prematurely or leaked when being hauled up. From the vertical distribution curves the following data are taken: (1) the interpolated values at standard depths, and (2) the depths of selected values of a given property at a number of stations. These data are necessary in order to construct vertical sections of the distribution of any property.
Besides the vertical distribution curves, it is common practice to prepare other plots of various kinds which serve either to detect errors or to bring out characteristic features of the data. One of the most common of these is the temperature-salinity curve (T-S curve), in which the corresponding values of temperature and salinity from a single station are plotted in a graph, with temperature and salinity as the coordinates, and are then joined by a curve in order of increasing depth. In any given area the shape of the T-S curve has a fairly definite form, and hence errors in observation may sometimes be detected from such a graph. Many other uses of graphs of this nature are discussed in chapter V. Similar constructions can be used for the other serial data whenever
The interpolated values of temperature and salinity at standard depths are obtained before the density, specific volume anomaly, and other calculations based upon these data are made (see chapter III). As a check upon the correctness of the data, it is often advisable to plot the vertical distribution of density (σι) and the specific volume anomalies for each station as functions of depth.
Various types of distribution diagrams may be prepared from the serial observations, many of which are shown elsewhere in this volume, particularly in chapter XV.
The interpretation of serial oceanographic observations depends so much on the nature of the data, the distribution of observations in space and time, and the characteristic features of the region under investigation that it is impossible to attempt to formulate any “system” of analysis. Only from a knowledge of oceanographic problems and of the significance of the various kinds of data can the most fruitful method of attack be decided upon in any given investigation. The development of new theories and new viewpoints may invalidate earlier conclusions, but the observational data remain valid if they have met the required standards of accuracy.
OBSERVATIONS OF TIDES
Observations of the tides are necessary to establish reference levels for depths and elevations, for reducing soundings to the local reference level (p. 10), for the preparation of tables of predicted tides for use in navigation, and to further the scientific study of tidal phenomena. The rise and fall of the sea surface associated with tidal movements is discussed in chapter XIV, where it is shown that in any locality the range of tide, character of tide, and time of tide with reference to the meridional passage of the moon must be obtained by observation. When adequate data are assembled, the tides at that locality may be predicted with great accuracy.
The essential data are a series of measurements of the elevation of the sea surface at certain time intervals referred to a standard time system, and from these measurements the rise and fall of the sea surface associated with the tide may be plotted as a function of time. In all such studies the elevations must be referred to one or more bench marks, which may or may not be connected with other points of tide observations by accurate leveling. Bench marks are necessary so that the
The simplest device for measuring the rise and fall of the tide on shore is a tide staff. A tide staff is a stout plank that is graduated in feet and tenths of feet or according to the metric system and which is securely fastened to some permanent structure such as a rocky cliff, cement dock, or piling. It must be of sufficient length to extend above the highest tide and below the lowest tide. The graduation must be adjusted with reference to the bench mark, so that if the staff is removed for repairs or replaced, the readings can be referred to a common base. If observations are made every hour, a complete marigram (tide curve) can be constructed, but in many cases observations are made only at certain selected times of the day, or only high and low water are measured. By comparison with the conditions at nearby stations, such random observations are sufficient to determine the character of the local tide. The tide staff is quite accurate in protected water where the waves are small, but on an open coast waves and swells may make it difficult to obtain accurate observations (Rudé, 1928).
In localities where waves cause difficulties, tape gauges are used for visual observations. A float is suspended in a well, commonly a large pipe with small openings below the lowest tide level, and attached to the float is a graduated tape that passes over a pulley, a counterweight being on the other end. The rise and fall of the surface due to waves is largely eliminated, and the tape may then be read with reference to some arbitrary level at appropriate time intervals (Rudé, 1928).
The principle of the tape gauge may be adapted for obtaining a continuous automatic record of the tide level. In the standard automatic gauge used by the U. S. Coast and Geodetic Survey (Rudé, 1928), the float, which is suspended in a well, is attached to a wire that turns a pulley mounted on a threaded rod. As the pulley turns, a carriage with a pencil moves back and forth along the threaded rod that is mounted at right angles to a clockwork-driven roller carrying a sheet of paper. The paper is driven ahead about one inch per hour, and the device thus traces the marigram automatically. Suitable reduction is obtained by varying the size of the pulley and the pitch of the threaded rod. An accurate clock makes a special mark every hour, and a fixed pencil traces a reference line. Short-period waves are largely eliminated because of the damping in the well, but seiches and disturbances of the sea surface lasting several minutes or more are recorded (p. 542). From the marigram the hourly heights and the levels and times of high and low water are easily read off. The standard gauge carries enough paper for one month, but the clocks must be wound once a week, and each day the
The devices so far described can be used only on shore or where some rigid structure extends above the sea surface. Many types of pressure-recording devices for use on the sea bottom have been designed. In some the pressure element only is placed below the sea surface, with the recording device on land; in others, such as those to be used far from shore, the recording device is an integral part of the instrument, which may be placed on the sea floor or anchored and left in position for a week or more. Such open-sea tidal recorders are not beyond the experimental stage. Descriptions of the various types may be found in the Hydrographic Review. The character of the tide over shoals in the open sea may be determined from an anchored ship by means of repeated wire or sonic soundings, if the bottom is flat enough to warrant such observations.
DEEP-SEA ANCHORING
Oceanographic vessels are sometimes anchored in deep water for periods ranging from part of a day to as much as two weeks for the purpose of measuring currents at subsurface levels and for obtaining repeated observations on the vertical distribution of properties at a single locality. Such observations are made to confirm the validity of currents computed from the distribution of density and to obtain measurements of the water movements associated with the tides, internal waves, and other periodic and aperiodic disturbances. Knowledge of the fluctuations in the vertical distribution of properties is valuable in establishing the significance of single sets of observations and in the analysis of the character of internal disturbances.
For the accurate measurement of currents a fixed point of suspension is required, but this condition cannot be fulfilled by a vessel anchored in depths of several thousand meters. Not only does the vessel swing in a relatively large arc, but it also tends to ride up on the cable and then fall away again. In addition, the anchor usually drags somewhat in the soft bottom. So far, it has not proved practical to anchor in deep water with more than one cable. In order to eliminate as far as possible the effect of the vessel's movements on the current measurements, the tension on the cable is noted, detailed records are made of the direction and velocity of the wind and surface currents, and numerous astronomic fixes are taken. The movements of the anchored vessel are generally not significant when making repeated serial observations to
The first vessel to be anchored successfully at great depths was the U.S. Coast and Geodetic Survey steamer Blake, which in 1888–1889, under the command of Lieutenant J. E. Pillsbury, carried out studies of the Gulf Stream (Pillsbury, 1891). The Blake was anchored at thirty-nine localities in depths as great as 4000 m, where currents were measured and subsurface temperatures were determined. Under the direction of Helland-Hansen the Michael Sars and Armauer Hansen have been anchored at great depths in the North Atlantic, and other vessels have since been anchored at great depths elsewhere. The Meteor (Spiess, 1932b), Willebrord Snellius (Perks, in Pinke, 1938), and Atlantis (Seiwell, 1940) have all anchored in depths greater than 4500 m for periods of from several hours to as much as two weeks. The greatest depth in which a vessel has been anchored is about 5500 m, at which depth the Meteor anchored for two days.
Because of the weight of the wire rope suspended in the water, extremely strong hoisting gear and deck fittings are required. The wire ropes are generally tapered multistrand steel ropes of a special nonkinking type. Some have hemp cores for part of their length. The ropes used on the Blake, Meteor, and Snellius had a diameter at the free end of about 3/8 inch, which increased to about 5/8 inch at the winch end. Near the anchor, manila rope, chain, or special cable is used to avoid kinking the wire rope on the bottom. Various types of anchors have been used, either singly or in pairs, which weigh between 400 and 500 lb. The anchors are either of standard patterns with somewhat enlarged flukes or they are of the mushroom type. The weight of the anchors is apparently not very important. The E. W. Scripps has anchored successfully in depths of about 1600 m using a Danforth anchor weighing only 40 lb. The hoisting is usually done with a steamor power-driven capstan head of large diameter, the wire rope being wound under tension on a winch drum. As the strains may exceed several tons, all fittings must be of stout construction. The wire rope is usually led through an accumulator to ease sudden strains, over a dynamometer, through a braking device, and then over a large pulley or roller mounted on the bow. A meter wheel is necessary to measure the amount of rope payed out. The equipment and its use are described in the references already cited.
When anchored in the strong current of the Gulf Stream, the Blake used a scope (ratio between the amount of wire rope payed out and the depth of water) of two or three, but for anchoring in the open sea scopes between 1.1 and 1.6 have been used. The smaller the scope, the less will be the movement of the vessel, but the surface current and the wind and sea will determine the needed scope.
CURRENT MEASUREMENTS
The ocean currents are complicated because, superimposed upon the major currents that transport enormous masses of water, there are irregular eddies that may reach to great depths, wind currents that are confined to the surface layers, and tidal currents or currents associated with internal waves which are present at all depths between the surface and the bottom but which change periodically. In many instances the major currents cannot be directly measured, but conclusions as to their directions and velocities must be based on the application of the laws of hydrodynamics to the observed distribution of density. The methods used in such studies are dealt with in chapter XIII. Here will be discussed only the methods for direct observation, and these can be conveniently classified into two groups: drift methods and flow methods. An excellent summary of all methods and a description of instruments used have been prepared by Thorade (1933).
In scientific literature the velocity of a current is given in centimeters per second (cm/sec) or occasionally in meters per second (m/sec), but in publications on navigation the velocity is stated in knots (nautical miles per hour) or in nautical miles per 24 hours. The term “knots” dates back to the time of the sailing vessels when the speed of the vessel was measured by chip log, log line, and sand glass. Along the log line distances from a zero mark were shown by short strings provided with knots. On the first string was tied one knot, on the second two, and so on. When the chip log was thrown overboard the log line would be pulled out and as the zero mark passed the rail the sand glass was turned. When the sand glass had run down, the log line was stopped and the number of knots on the nearest string were counted. The sand glass and the distances between strings were adjusted to give the speed of the vessel in nautical miles per hour; thus the number of knots gave the speed in this unit—that is, in “knots.”
The direction is always given as the direction toward which the current flows, because a navigator is interested in knowing the direction in which his vessel is carried by the current. The direction is indicated by compass points (for example, NNW, SE), by degrees reckoned from north or south toward east or west (for example, N 60°W, S 30°E) or in degrees from 0° to 360°, counting current towards north as 0° (or 360°) and current towards south as 180°.
Drift Methods
Information as to the general direction of surface currents is obtained from the drift of floating objects such as logs, wreckage from vessels, and fishermen's implements. Thus, glass balls used by Japanese fishermen and wrecked Chinese junks are sometimes found on the west coast
More than a century ago, in order to overcome such uncertainties, drift bottles were introduced. These are weighted down with sand so that they will be nearly immersed, offering only a very small surface for the wind to act on, and they are carefully sealed. They contain cards giving the number of the bottle, which establishes the locality and time of release, and requesting the finder to fill in information as to place and time of finding and to send this information to a central office.
In order further to insure reduction of the direct effect of the wind, drift bottles have sometimes been provided with a kind of drift anchor—for example, a cross-shaped piece of sheet iron suspended about 1 m below the bottle. In other instances, two bottles have been used, one of which has been weighted so much that it is carried by the other, the connecting wire between the bottles being about 1 m long. Still other experiments have been conducted with two bottles, one containing a weak acid which in a given length of time corrodes a metal stopper, thus permitting the sea water to fill the bottle. When this takes place, the bottles sink to the bottom, where they are held by a piece of sheet metal that acts as an anchor. This device has been used in the shallow waters of the North Sea, where bottom trawls are used extensively by fishermen, who recover many of the bottles.
The interpretation of results of drift-bottle experiments presents difficulties. In general, a bottle has not followed a straight course from the place of release to the place of finding, and conclusions as to the probable drift must be guided by knowledge of the temperature and salinity distribution in the surface layers. Fairly accurate estimates of the average speed of the drift can be made if the bottle is picked up from the water, or if a special drift bottle is brought up from the bottom. Bottles that are picked up on frequented beaches can also be used for estimating the speed of the drift. Tait's conclusions (1930) from the
Drift bottles have been used successfully for obtaining information as to surface currents over relatively large ocean areas, such as the equatorial part of the Atlantic Ocean (Defant, 1929, p. 34) and the seas around Japan (Uda, 1935). They have supplied numerous details in more enclosed seas like the English Channel and the North Sea (Fulton, 1897; Carruthers, 1930; Tait, 1930), but have proved less successful off an open coast (Tibby, 1939).
The drift method can also be used for obtaining information as to currents in a shorter time interval. The currents derived from ships' records are determined by this method (p. 428) and give the average surface current in twenty-four hours or multiples of twenty-four hours. From an anchored vessel, say a lightship, the surface current can be determined either by a chip log (Bowditch, 1934, p. 11) or by drift buoys, below which, in general, there is a “current cross” acting as a sea anchor. This type of drift buoy was used on the Challenger. The latter methods give nearly instantaneous values of the surface currents at the place of observation.
Near land the methods can be elaborated in such a manner that the drift of a body can be determined in detail over long distances and long periods. A drifting buoy can be followed by a vessel whose positions can be accurately established by bearings on known landmarks, or the buoy can be provided with a mast and the direction to the buoy can be observed. Its distance from a fixed locality can then be measured by a range finder. Both methods have been used successfully. The latter can also be employed in the open ocean by anchoring one buoy, setting another buoy adrift, and determining the bearing of and the distance to the drifting buoy from a ship that remains as close as possible to the anchored buoy.
Still another drift method has been used with advantage in order to determine the ice drift in shallow waters out of sight of land. The method consists in letting a weight drop so rapidly to the bottom that it sticks in the bottom mud. The time and the length of wire rope payed out are recorded, and then more wire rope is payed out according to the drift of the ice floe to which the vessel is tied up. After a given length of
Flow Methods
From an anchored vessel or float, currents can be measured by stationary instruments past which the current flows, turning a propeller of some type or exerting a pressure that can be determined by various methods. The advantage of these instruments is that observations need not be limited to the currents of the surface layers but can be extended to any depth. The obvious difficulty is to retain the instrument in a fixed locality so that the absolute flow of the water may be measured and not merely the flow relative to a moving instrument. In shallow water a vessel can be anchored so that the motion of the vessel is small enough to be insignificant or of such nature that it can be eliminated. In deep water, current measurements were first made from anchored boats, but in later years the technique of deep-sea anchoring has been advanced (p. 361) to such an extent that vessels like the Meteor, Armauer Hansen, and Atlantis have remained anchored in depths of from 4000 to 5500 m for days and weeks. In other instances, relative currents have been measured from slowly drifting vessels.
Maintaining a vessel at anchor for a long time is expensive, and devices have therefore been developed for anchoring automatic recording current meters that can be left for weeks at a time (p. 370). Measurements of currents very close to the sea bottom cannot be made safely from an anchored vessel, no matter how securely it is kept in position, because an instrument suspended from the vessel cannot be retained at a constant distance from the bottom owing to the motion due to swells and tides. This difficulty was first overcome by Nansen, who lowered a tripod to the sea bottom and suspended a current meter from the top of the tripod. The same method was later used by Stetson (1937), Revelle and Fleming (p. 480), and Revelle and Shepard (in press). The latter suspended three current meters from the top of the tripod and thus were able to obtain simultaneous measurements of currents at three levels within less than 2 m of the bottom.
Current Meters
Current meters differ in design, but all propeller or cup instruments have some device for counting the number of revolutions of the propellers or cups in a given time interval, a vane for orienting the meter in the direction of the current, and a more or less complicated mechanism for recording this direction, either relative to the magnetic meridian (by compass) or relative to some fixed plane (bifilar suspension). Instruments that measure the pressure may not have a vane, because the
Use of a compass for determining the direction of the current has the disadvantage that near a steel vessel a compass needle will be greatly influenced by the ship's magnetism. The deviation due to the ship's magnetism may be as much as 180°; that is, off the side of a vessel a compass needle may be completely reversed. This deviation, which decreases rapidly with depth, depends upon the heading of the ship, the latitude, and the depth of the current meter. It changes with time because a great part of the ship's magnetism is not permanent. It is a hopeless task to determine this deviation for all headings of the ship and all depths of measurement, and therefore a compass should not be used for observing directions from a steel vessel at depths of less than 50 m. Even from wooden vessels directions by compass have to be carefully examined, particularly if the depth is less than 20 m. For the upper layers, a bifilar suspension is recommended with an arrangement for recording the direction of the current relative to the orientation of the bifilar frame, which again can be determined by means of the ship's heading. A compass instrument can be used near the surface from an anchored boat, particularly if a manila rope is used for anchoring and not a steel rope or an anchor chain.
All propeller and cup instruments suffer from the disadvantage that drifting material may impede the motion of the screw or stop it completely. Instruments designed to be left in position for a long time should be checked at frequent intervals to insure perfect functioning. It should also be borne in mind that jellyfish or similar organisms may be caught on the wire rope and prevent a messenger from passing.
Owing to its simplicity and reliability, the Ekman current meter (Ekman, 1905, 1932) has been and still is widely used. This instrument is described in detail below. The greatest disadvantage of the Ekman meter is that only one instrument can be attached to the wire and that it has to be hauled up to be read after each period in which the propeller
Continuously recording current meters have obvious advantages, but they are complicated and expensive. The recording device may be mechanical, requiring a clockwork that will function reliably in sea water, or electrical, requiring contacts that are insulated from the sea water, or photographic, requiring a watertight chamber which can withstand the pressure and in which the photographic equipment is enclosed. Detailed descriptions of various designs are given by Thorade (1933). Here only a brief summary is given of the most important features of instruments that have been or are in use.
Ekman Current Meters (Ekman, 1905, 1932). The essential parts of this instrument are the propeller, the revolutions of which are recorded on a set of dials, the compass box, with the device for recording the orientation of the meter, and the vane which orients the instrument so that the propeller faces the current (fig. 88). The free swing of the instrument is ensured by mounting it in ball bearings on a vertical axis. The wire for lowering is fastened to the upper end of this axis, and a suitable weight is attached below the axis. The instrument is balanced in water so that the axis is vertical. The carefully balanced propeller, with four to eight thin, light blades, runs inside a strong protective ring but can easily be removed for inspection or transportation. The axis of the propeller runs with tantalum points on agate bearings. Inside the protective ring is a lever that can be operated by messengers. With the lever in its lowest position the propeller is arrested, and in this state the instrument is lowered. When the desired depth is reached, a messenger weight is dropped, pushing the lever up to its middle position and releasing the propeller, the turns of which are recorded on a set of dials. After a number of minutes a second messenger weight is dropped which pushes the lever up to the highest position and stops the propeller. In later types the propeller is also shielded in front when the instrument is lowered in order to prevent fouling of the propeller by such organisms as medusae. This front shield is opened by the first messenger.
The direction of the current is recorded by an ingenious device that is simple and reliable. A tube extends from above the dial box to a
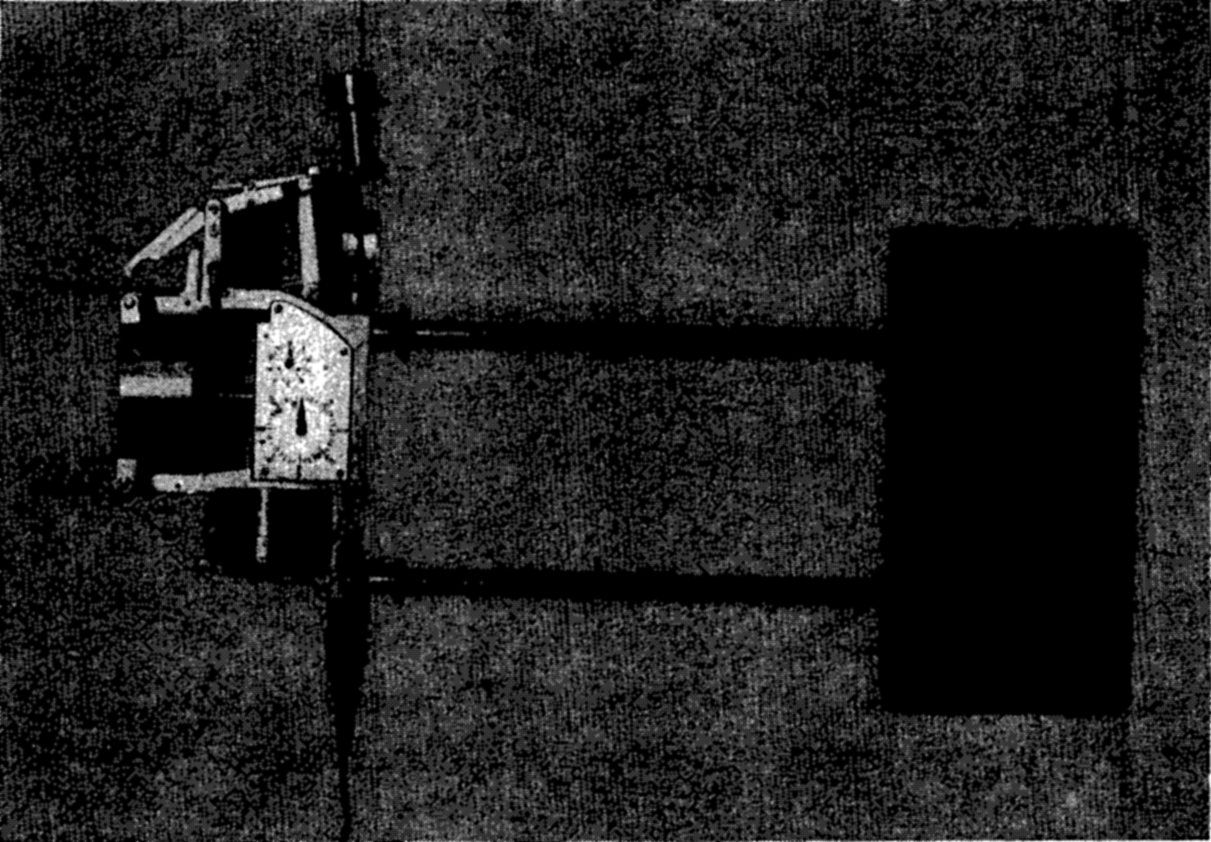
The Ekman current meter. Messenger, dials, and compass box are seen. Propeller is hidden by protective ring.
[Full Size]
Ekman Repeating Current Meter (Ekman, 1926). In this instrument the propeller is released and stopped by messengers. When the propeller is stopped, three numbered balls are released from a container. One ball drops down into a compass box, giving the direction of the current at the time the propeller was stopped, and two balls are guided into other slots by the position of dials turned by the propeller. From the slots into which the balls fall, the positions of the dials can be found and thus the number of revolutions of the propeller can be obtained. The messengers are designed to split when they strike the instrument, and the two parts are caught in a container. The operation can be repeated forty-seven times, when the store of numbered balls is exhausted.
Carruthers Residual Current Meter (Thorade, 1933). This instrument is designed for giving the residual current over a long period of time. It has no device for directly recording the revolutions of the cups, but after a certain number of turns a ball is released and drops down into a compass box similar to the compass box of the Ekman meter. The velocity is obtained from the number of balls released. The balls are supplied from a large box containing more than 22,000. At the end of the period of measurement, which may comprise one or more days or even weeks, the number of balls in the slots of the compass box are counted. From these data and the calibration results the average direction and velocity can be found.
Böhnecke Mechanical Recording Current Meter (Thorade, 1933). In this current meter the propeller drives a set of horizontal dials with raised numbers on their vertical rims. A similar dial is attached to the magnet of a compass. A strip of tin foil passes the vertical rims of these dials, being rolled by clockwork from one spool to another. At intervals of five or ten minutes a hammer presses the tin foil against the raised numbers on the rims of the disks, thus recording the positions of the dials, which are turned by the propeller and the magnets of the compass. The instrument can aIso be suspended in a bifilar frame and the direction relative to the orientation of that frame can be recorded. The instrument has not been widely used, probably because it is difficult to find material for the spring in the clockwork, which will be exposed to sea water.
Witting Electrical Recording Current Meter (Witting, 1923). In this instrument the axis of a wheel that is turned by a propeller carries an eccentric disk operating a fork-shaped lever. Through a semiwatertight connection, one part of the fork is brought into a circular box filled with petroleum, where it raises or lowers a magnet. In raised position the magnet is free to swing, and no electrical current passes through the system. In lowered position the frame to which the magnets
Sverdrup-Dahl Electrical Recording Current Meter, Compass Type (Sverdrup And Dahl, 1926; Sverdrup, 1929). This instrument is similar to the Witting current meter except that the contact rings are not enclosed in a semiwatertight box, but are arranged at the top of an inverted brass cylindrical container that serves as a diving bell. Before the instrument is lowered, petroleum is introduced into a vessel in the cylinder. When lowering, the petroleum floats on top and protects the contacts against the sea water, which, with increasing pressure, rises higher and higher. The magnet rests on a pin that is lifted and lowered as the propeller turns. The electric wiring differs somewhat from Witting's, but the record is similar.
Sverdrup-Dahl Electrical Recording Current Meter, Bifilar Type. In this current meter the vane of the instrument turns a sliding contact that rests against a resistance ring rigidly connected with the bifilar suspension. The electric circuit is closed and opened by a key operated by the propeller, remaining closed for about eighty revolutions of the propeller and open for about twenty. The electric contacts are arranged at the top of a “diving bell,” and a recording milliammeter serves as recording instrument. The last-named three instruments require only one insulated conductor, the suspension wire serving as a second conductor.
Ott Electrical Recording Current Meter (Thorade, 1933). In this meter the current velocity is recorded on a chronograph on which a mark is made electromagnetically when the propeller has completed a given number of turns. The direction is not recorded continuously, but by a somewhat complicated arrangement it is shown on a dial whenever the observer on board ship presses a button. All electrical contacts are enclosed in petroleum-filled chambers. Two insulated conductors are required.
Rauschelbach Electrical Recording Current Meter (Rauschelbach, 1929). This instrument is designed for bifilar suspension. The velocity is recorded on a chronograph by contacts made for every
Pettersson Photographic Recording Current Meter (O. Pettersson, 1913, H. Pettersson, 1915). The propeller of this meter is mounted on a vertical axis below a watertight cylindrical chamber. Outside this cylinder the propeller, through a reduction gear, turns a strong magnet which by induction turns a similar magnet in the cylinder. The magnet in the cylinder carries a disk with a transparent division along the rim. The compass carries a smaller disk with transparent division, the two disks being concentric. A short cylinder of soft iron shields the compass magnet from the magnets rotated by the propeller. Every half hour the positions of the two disks are photographed on a film that is advanced by clockwork. The clockwork also turns on and off a small electric bulb, the power for which is supplied by a storage battery. The meter is designed for suspension below a buoy that can be anchored 10 m or more below the sea surface and left for two weeks.
Idrac Photographic Recording Current Meter (Idrac, 1931). The cups of this instrument are mounted on a vertical axis and operate an electric contact which is placed at the top of an inverted cylindrical container similar to that used in the Sverdrup-Dahl meter. When the electric circuit is closed, a lamp is lighted and a mark is made on a film that is advanced at a constant speed by clockwork. The clockwork and the camera are enclosed in a watertight cylinder, and a storage battery is enclosed in a similar cylinder. The current velocity is obtained from the number of marks on the film in one hour. The direction is recorded continuously. Attached to the magnet of the compass is a black disk on which are marked two white concentric circles connected by a white spiral. The spiral rises from the outer to the inner circle in exactly 360 degrees. The camera is mounted above the disk and is provided with a narrow slit through which a very narrow strip of the disk is photographed, the two white circles and the white spiral appearing as points. If the orientation of the meter relative to the magnetic meridian remains constant, all points will produce straight lines on the moving film, but, if the meter turns, only the two circles give straight lines and the spiral gives a curve from which the orientation of the meter—that is, the direction of the current—is obtained.
Winters Photographic Recording Current Meter (Thorade, 1933). The Winters meter is constructed on principles similar to those of the Pettersson meter, but photographs of the counting device and of the position of the compass card are obtained at intervals of five minutes.
Nansen Pendulum Current Meter (Nansen, 1906). This instrument is designed for mounting on a tripod and for measuring very weak currents near the sea bottom. A light pendulum swings above a slightly concave disk which is carried by a magnet and which is covered by graduated waxed paper. At short intervals of time a clockwork lowers the pendulum and a fine stylus attached to its bottom marks the paper, indicating the direction and velocity of the current.
Jacobsen's Bubble Current Meter (Jacobsen, 1909). This current meter is designed for use from such vessels as lightships. It employs no propeller or compass, but measures the magnitude and direction of the deflection of a pendulum relative to the ship from which the pendulum is lowered into the water. Cylinders open at both ends are used as pendulums. The line from the cylinder is fastened to a rod, the orientation of which as to inclination and plane of inclination is observed by a bubble in a segment of a sphere.
Buchanan-Wollaston Mechanical Recording Current Meter (Buchanan-Wollaston 1925, 1930). In this instrument the current velocity is recorded by the pressure exerted by the current against two perforated disks which always remain in a vertical position and which are made to face the current by a vane. The recording unit is enclosed in a watertight cylinder that is mounted horizontally and that turns around a horizontal axis when pressure is applied to the two perforated disks. The turning of the cylinder is recorded, and at intervals of twenty minutes the direction by compass is indicated. The instrument has the advantage that it carries no screw to be clogged by drifting objects, but it is not sensitive to weak currents, since the minimum velocity that can be recorded is about 12 cm/sec.
Analysis of Records of Currents
Different methods of representing surface currents are discussed on pp. 427–430. For currents of a periodic character, it is convenient in most cases to compute the north-south and east-west components of the currents or the components referred to some other coordinate system, say parallel to and at right angles to the coast. The components can readily be subjected to harmonic analysis or to other forms of statistical treatment, subsequent to which the results can be represented in a simple manner (see fig. 145, p. 573). Harmonic analysis has been widely applied and is a very useful tool (see Thorade, 1933).
COLLECTION AND ANALYSIS OF BIOLOGICAL SAMPLES
Biological investigations in any area consist essentially of two parts: (1) descriptive and (2) analytical.
The descriptive part is concerned chiefly with determining the kind of the organisms present and their phylogenetic relationships, and with establishing their geographic and bathymetric distribution. This phase of marine biology was the first to develop historically, and is naturally the first in the investigation of new areas. The relative volume of strictly descriptive work gradually diminishes as the various groups of organisms and their distribution become known, but the application of the results will always be a part of biological studies.
The second, or analytical, phase is concerned above all with the factors operative in the development and distribution of the populations and with the biologic economy of the inhabitants as related to the inorganic and organic factors of the environment. Some of the factors operative in nature can be studied under controlled experiments in the field or in the laboratory, but in any event the information gained must be applied to an interpretation of the complex environmental conditions as they affect the lives of organisms found in the sea. Therefore, the collection of representative samples of plants and animals in nature and the analysis and interpretation of these samples become items of prime importance in order that true correlations can be found.
From the outset it must be realized that at best any sample of organisms collected from the sea is only a minute portion of a population that may occupy a tremendously large area of the sea or only a restricted portion thereof, and that the concentrations are often very irregular. It is only through the collection of numerous samples in various areas and seasons that a true picture of the members of the population—their distribution, life cycles, and interrelations—can be acquired, The nature, size, motility, and habitat of the organisms determine the type of collecting equipment to be used.
Collection of Benthic Organisms
In the intertidal region, only simple equipment is needed, but for collecting in deep water various types of dredges and grabs are used. A dredge (fig. 89b) consists essentially of a heavy rectangular or triangular iron frame to which is attached a baglike fish net of cotton or wire web to retain the organisms. The dredge is dragged on the bottom by means of a wire cable operated from a power winch aboard a slowly movingship. The size of dredges used varies greatly, depending upon the equipment available for their manipulation aboard ship. A practical size for use on a vessel 15 m or more in length and in water of moderate depth has a beam of about 1 m. Only stationary or slowly moving organisms are caught; microscopic forms are included if they are incidentally attached to larger animals and plants or in sediment that has not been completely washed through the meshes of the net during the ascent of the dredge. The
Illustrative of equipment for quantitative collecting of benthic life is the Petersen grab, or bottom sampler, developed by Petersen (1918) for quantitative investigation of benthic animals in relatively shallow waters. It consists of a pair of very heavy metal jaws that are held open during the descent (fig. 89a). When the grab strikes the bottom, the slackened cable releases the tension on a clutch that holds the jaws open, and, when the cable is again drawn tight by the winch aboard ship, the jaws snap shut by their own weight and enclose the material, including the sessile organisms, covering a measured area, usually 0.1 m2, of the bottom over which the open jaws descended. The organisms caught are screened from the bottom sediments, classified, and counted
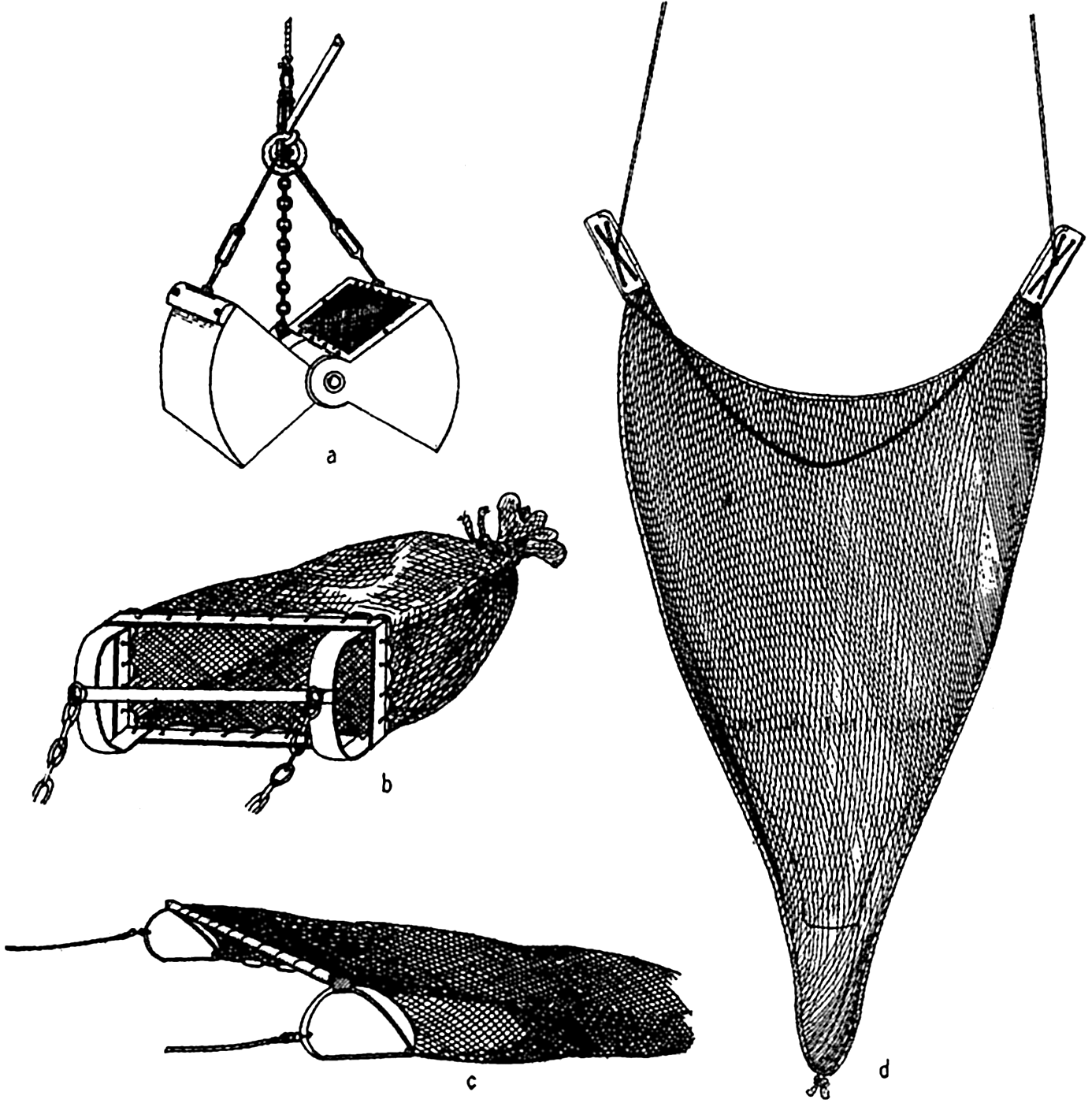
Gear for biological collecting: a, Petersen grab; b, dredge; c, beam-trawl; d, otter-trawl.
[Full Size]
Small snappers and coring devices (figs. 84, 85) are useful in collecting quantitative biological samples only of microscopic organisms such as foraminifera and bacteria.
Collection of Nekton
In commercial fishing, diverse methods involving nets, trawls, traps, hooks, and harpoons are used, depending upon the animals sought, but we shall here consider only the trawl, which has been much used in collecting deep-water animals for scientific research. The beam trawl (fig. 89c) is constructed somewhat like the dredge, but the frame does not form digging and scraping edge and it may have a much larger opening, up to 15 m or more; since it is not designed to dig into the bottom, it may be towed at greater speed and thus catch the faster moving animals—shrimps, fishes, and so forth—that live on or near the bottom.
For pelagic trawling the otter trawl is used mostly. The opening to the web sack is kept distended, not by a rigid beam, as in the beam trawl, but by means of otter boards attached to opposite sides of the opening. Upon being towed, these boards are forced apart by the resistance of the water (fig. 89d). The span of the opening may be 20 to 26 m and the net may be 40 m long. A small otter trawl was employed successfully by the Michael Sars in fishing at depths as great as 5160 m.
The ring trawl is essentially a large, relatively coarse plankton net attached to a strong ring of large diameter and provided with a towing bridle (see below).
Collection of Plankton
A great variety of nets and other equipment has been used in obtaining samples of the phyto- and zooplankton. The plankton tow net was apparently first introduced by Johannes Müller in 1846 and has found the widest use of all plankton-collecting devices.
The plankton net consists of a filtering cone attached to a metal ring by which the net is towed through the water. Detailed instructions for cutting patterns for ordinary nets are given by Seiwell (1929). The filtering material forming the net is usually some grade of silk bolting cloth of the type used for sifting flour. It is numbered from 0000 to 25, depending upon the number of meshes per linear inch. The strands are so woven that the aperture size is not readily changed; hence they differ from those in ordinary weaving, where the strands cross each other alternately without a binding turn (fig. 90). The dimensions of the
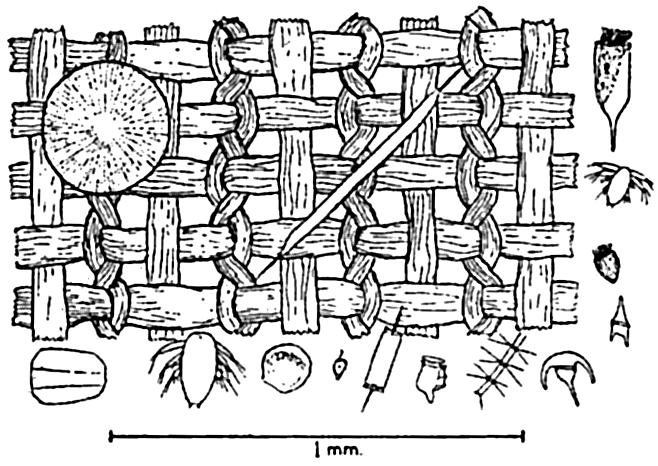
Enlarged camera lucida drawing to show weave of No. 20 bolting cloth, together with aperture size compared with the size of some common plankton organisms drawn to the same scale. Organisms, horizontal row: Coscinodiscus granii, nauplius Stage III Acartia; early clam larva, Prorocentrum micans Ditylum, Dinophysis, Chaetoceros, Ceratium tripos, Vertical row, down: Favella, nauplius Stage I, Acartia, Stenosomella, Ceratium furca. On cloth: Coscinodiscus wailsii and Rhizosolenia semispina, two unusually large diatoms.
[Full Size]
At the cod, or tail, end of the net a detachable jar, vial, or small strainer of some type is placed to receive the concentrated tow.
A continuous plankton recorder has been developed by Hardy (1936) for towing behind a ship while under way at full speed. The machine is essentially a torpedo-shaped tube, circular or rectangular in cross section, and about 1 m long. The front end is provided with a small hole for entrance of water as the machine is hauled forward. This opening leads to a wider tunnel across which a long band of silk gauze is slowly wound from one spool to another and through which the water must pass before its exit by way of a hoIe at the rear end of the tube. The gauze, with the plankton screened from the water, is continuously rolled onto the storage spool. The winding is done by a propeller outside of the machine, and thus the speed at which the band is wound within the machine is controlled by the speed with which the whole machine is drawn through the water and is therefore in direct relation to the
Silk No. | Meshes per inch | Size of aperture (mm) | Silk No. | Meshes per inch | Size of aperture (mm) |
---|---|---|---|---|---|
0000 | 18 | 1.364 | 10 | 109 | 0.158 |
000 | 23 | 1.024 | 11 | 116 | 0.145 |
00 | 29 | 0.752 | 12 | 125 | 0.119 |
0 | 38 | 0.569 | 13 | 129 | 0.112 |
1 | 48 | 0.417 | 14 | 139 | 0.099 |
2 | 54 | 0.366 | 15 | 150 | 0.094 |
3 | 58 | 0.333 | 16 | 157 | 0.086 |
4 | 62 | 0.318 | 17 | 163 | 0.081 |
5 | 66 | 0.282 | 18 | 166 | 0.079 |
6 | 74 | 0.239 | 19 | 169 | 0.077 |
7 | 82 | 0.224 | 20 | 173 | 0.076 |
8 | 86 | 0.203 | 21 | 178 | 0.069 |
9 | 97 | 0.168 | 25 | 200 | 0.064 |
A smaller and simpler device known as the plankton indicator has been developed (Hardy, 1936) mainly for use by herring fishermen in determining the general type of plankton in the water before casting their nets. It consists of a tube 56 cm long, 8.9 cm in diameter, and with both ends tapering to 3.8-cm openings. Across the lumen of the tube a small disk of bolting cloth is placed for screening out the plankton as the tube is drawn forward through the water at full speed of the boat. The disk can be quickly removed and a clean one inserted, thus allowing frequent direct gross examinations to determine whether patches of phyto- or zooplankton are being traversed (see p. 907).
Zooplankton. Attempts to standardize the nets of various types have led to a comparison of the “catching power” of several common nets as compared to the Hensen egg net, which for basis of comparison is rated as having a catching power of 1.0 (Künne, 1933). In comparison with the Hensen net, the Nansen net was found to have a catching power of 0.87, or, in other words, under comparable conditions quantitatively it caught nearly the same number of animals in the same proportion as the Hensen net. The standard net, which is a modified Nansen net, caught much less, its catching power being only 0.1, while the Helgoland larvae net was rated at 4.1. It should be emphasized that the catching power refers only to the relative capacity of each net to catch the animals
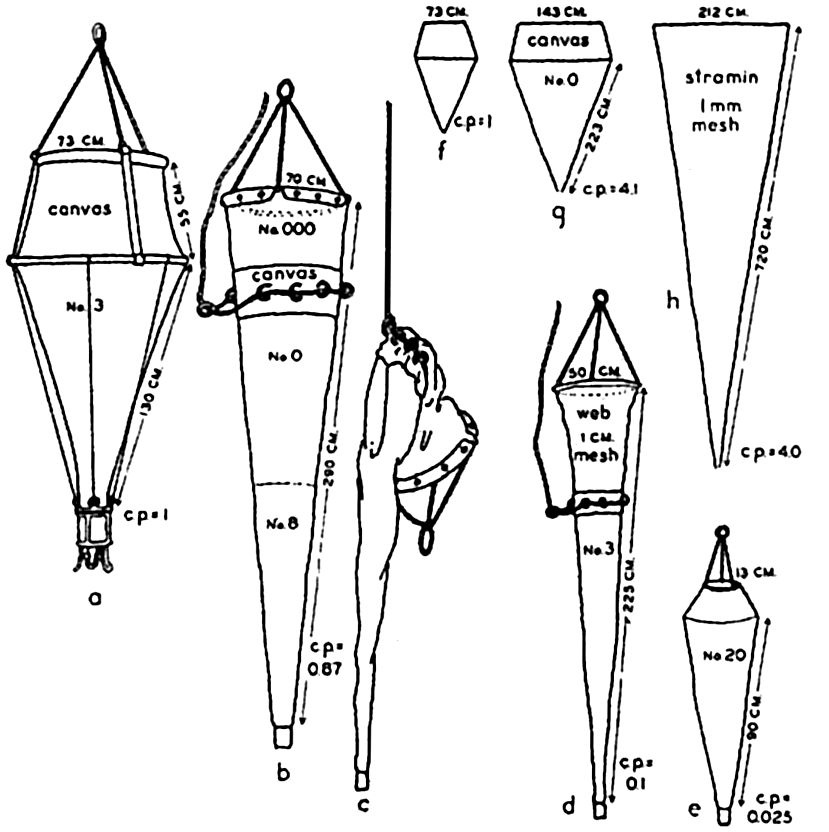
Types of plankton nets, their length, mouth diameter, type of filtering material, and relative catching power (c.p.), as explained in text: a to e drawn to same scale; f to h to a reduced scale. a, Hensen egg net; b, Nansen net open; c, Nansen net closed; d, standard net; e, medium Epstein net; f, Hensen egg net; g, Helgoland larva net; h, large vertical net of stramin.
[Full Size]
The truncated nets, illustrated especially by the Hensen egg net and the Apstein net, have a reduced opening at the head end in order to increase the ratio of the filtering area of the net to its mouth area and at the same time, by means of the canvas head piece, to minimize backwash while the net is being towed through the water.
Some nets, illustrated by the Nansen and standard nets, may be closed by means of a messenger that activates a mechanism (fig. 92) releasing the bridle lines and thus causing the strain to fall upon the puckering line, which, at any desired depth, closes off the head end of the net (fig. 91b,c). Thus it is possible to obtain a sample of the plankton population at any subsurface level without contamination by the organisms living in the overlying water layers.
Single nets are sometimes hauled through the water horizontally at the desired depth, or a series of nets may be placed at given intervals on the wire in order to sample several water strata at once. Commonly a net is payed out to a given depth, and, after a period of fishing at this level, it is hoisted successively to a series of higher levels to fish for the same length of time at each before being hauled in, thus obliquely sampling several strata with one net. In the above methods of sampling, it is difficult to obtain an estimate of the amount of water that has been filtered. A more reliable estimate of the amount of water filtered is gained if the net is towed vertically instead of horizontally or obliquely.
In vertical hauls the net, with the cod end tied to a weight suspended by long lines from the top ring, is lowered to the desired depth and then raised vertically, filtering a column of water the length of the course traversed. There is always a considerable amount of backwash, however, resulting from the resistance of the net, especially if it is constructed of fine mesh or has become partially clogged by the plankton. Hence somewhat less water has been filtered than would have gone through an open ring of the diameter of the net mouth. Therefore, when using the area of the net opening as the cross section of the water column filtered, the figures obtained for the number of organisms caught are always minimal ones. As a rule, also, it is not possible to haul the net exactly vertically, since the boat from which it is operated usually drifts more or less, resulting in a haul somewhat longer than the true vertical. There appears to be no sure method of avoiding these types of collecting errors inherent in the use of ordinary nets. Sometimes a recording meter is placed in the mouth of the net so that the water passing into the net activates a small propeller attached to a counting mechanism. When properly calibrated, the number of revolutions of the propeller is taken to indicate the amount of water having passed through the net. From this the number of organisms per unit volume of water, usually per liter or per cubic meter, can be calculated, but, owing to unavoidable irregularities in filtration or analysis, the figures obtained are at best usually only
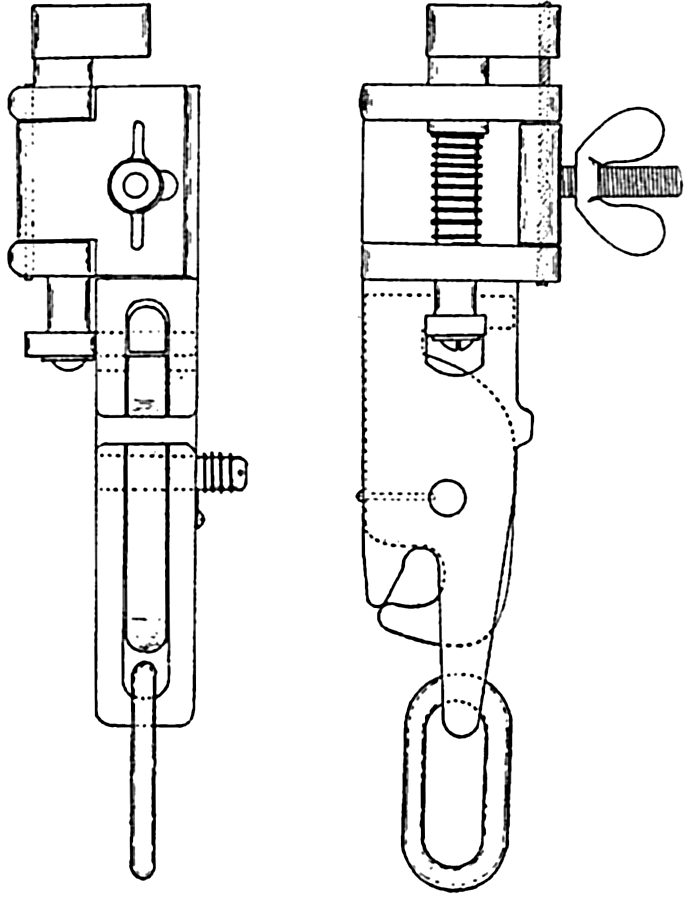
A tripping mechanism for closing nets and similar apparatus at subsurface levels; back and side views.
[Full Size]
Total filtration and exact analysis, of course, will be more nearly realized in sparsely populated waters, while in dense populations or in the presence of gelatinous objects, such as jellyfishes or cast-off appendicularian vestments (“houses”), the errors must become greater. Coarse-meshed nets provided with a water-measuring device represent the most ideal combination for collecting, but they are highly selective and can be used only where such selection is desirable on the basis of size—for example, in the study of large fish eggs or larvae. For a statistical study of the variations in catch of plankton nets the reader is referred to Winsor and Clarke (1940), and the relevant works included in their bibliography.
In an attempt to overcome the uncertainties inherent in net collecting of plankton, various devices (bottles and buckets) have been designed to entrap a definite amount of water together with its plankton population from a definitely known depth. Pumps operated from aboard ship and provided with a long hose, the intake of which can be lowered to the desired depth, have been used successfully, especially in fresh water, for sampling the population at depths down to about 75 m (Birge and Juday, 1922). Known amounts of water from a series of depths can in this way be filtered through fine nets, as the water is delivered from the pump, which is also provided with a water meter. These methods are excellent for some purposes, but are also subject to grave limitations, since they are useful only for inactive or relatively slowly moving organisms, when abundant and rather evenly distributed, such as diatoms, dinoflagellates, protozoa, and sometimes larvae. Fast moving, sparse, and irregularly distributed forms are not likely to be caught with sufficient regularity to give significant data. The ease with which such samples can be taken makes more samplings possible, and this advantage overcomes somewhat the adverse features.
Except for special studies, the zooplankton caught must be preserved in the field. Usually a 4 per cent solution of formaldehyde (preferably neutral) is used for this purpose. The collecting data on the label, placed inside the jar, should include serial number, date, hour, station number, depth sampled, and type of net used and how operated. The laboratory analysis of the samples usually consists of identifying and counting all of the desired species occurring in an aliquot portion of the well-mixed sample. From this is computed the total number of the selected species occurring in the whole sample. Finally, the volume of water filtered by the net in taking the sample having been approximately determined, the population is reported as the number of different
Phytoplankton. The regular Nansen hydrographic water bottle (fig. 87) is much used for collecting phytoplankton samples, or, if larger samples are desired, the Allen bottle (fig. 93), with a capacity of 5 l, can be used. When the Nansen bottle is employed, samples from certain levels, usually 1, 10, 25, 40, 75, 150 m or more, are tapped off directly into as many citrate bottles, neutral formaldehyde preservative is added, and the bottles are stored for examination in the laboratory. When the collection has been made with an Allen bottle (or similar large collector) at similar depths, the catch is immediately concentrated in a small volume of water (100–150 ml) by filtering through a small net of No. 25 bolting cloth. The sample is then preserved as above for study in the laboratory.
When the sample has not been concentrated in the field, the laboratory analysis consists first of centrifuging a measured portion (say 10 to 50 ml, depending upon the abundance of plankton) of the well-shaken sample. The clear fluid is withdrawn by means of a pipette, and the organisms that have been thrown down in the centrifuge tube are removed, together with the remaining fluid, to a cross-ruled glass slide. They are then covered by a cover slip, and the organisms are identified and enumerated under a compound microscope (Gran, 1932). The numbers obtained form tbe basis for calculating and reporting the
If the sample was concentrated in the field by filtering, an aliquot portion of the concentrated sample is placed in a Sedgwick-Rafter counting cell holding 1.0 ml of the sample, and the organisms are examined and reported as above.
The phytoplankton population may also be conveniently analyzed chemically by extracting the yellow pigments with acetone and reporting the population in numbers of plant-pigment units. In this analysis, the diatoms are filtered from a known quantity of water, and the pigments are then extracted with a measured volume of acetone. The tinted acetone resulting is compared colorimetrically with an arbitrary standard prepared by dissolving 25 mg of potassium chromate and 430 mg of nickel sulphate in 1 l of water. One milliliter of the standard solution is equivalent to one “pigment unit.” For a fuller discussion of this method and of other means of estimating phytoplankton production through utilization of plant nutrients and through oxygen production and consumption, see chapter XIX.
Bacteria. The collection of bacterial samples presents a very special problem, since they must be sterilely taken. In fig. 94 is shown a collecting bottle in the sampling frame with a combination glass and rubber filling tube. This tube is so designed that when the glass section is broken, the rubber part, which is under tension, projects the distal end of the tube, with the intake orifice, at some distance from the bottle and other equipment (ZoBell, 1941). The sea water entering the sterile bottle is thus free from contamination by bacteria that might otherwise be forced in from the surface of the sampling equipment. The frame, with the sampling bottle in place, is attached to the cable, and, at the desired depth, a messenger trips the tube-breaking device. Since the bottles are lowered empty, the depth at which samples can be taken by this means is limited to water pressures that will not break the bottle or force in the stopper. Experiments using rubber bottles to overcome these difficulties are now in progress. Laboratory analysis of the sample collected consists of plating out a known portion of the sample on nutrient material and noting the maximum number of colonies that grow, each
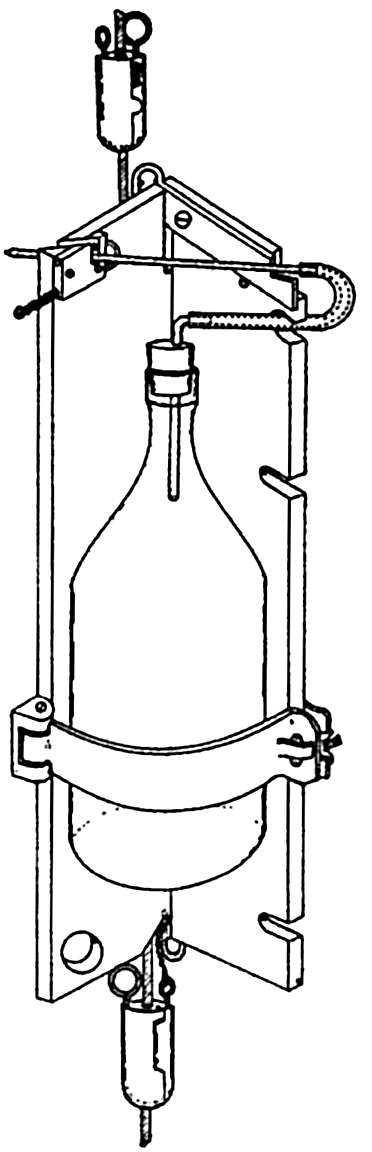
Bacteria sampling bottle in frame attached to cable. The upper messenger breaks the glass intake tube and releases the second messenger when samplers are used in series.
[Full Size]
The bacterial population in collected water or mud samples changes very rapidly, making it necessary to plate out the samples as soon as they are obtained in the field in order to obtain the most reliable estimates of the numbers of bacteria in situ. The type of culture medium used is of vital importance in obtaining a growth response from the maximum number of viable bacteria in the sample. Only media made up with sea water are effective (ZoBell, 1941).
The bacteria from bottom sediments are obtained by sterilely removing a sample of the undisturbed material in the center of a core taken with some type of coring device (p. 345).
Interpretation of Plankton Observations
In the interpretation of field observations on plankton, it must be remembered that the different requirements of separate species lead to a more or less complete change of the elements in the population when external factors, especially temperature and nutrients, become altered in the water mass inhabited. When such a biological change takes place within a rather well-defined water mass, whether moving or stationary, we may speak of it as an individual population succession. This must not be confused with a change of population resulting from a sequence of distinct water masses flowing with their distinct populations into a given geographical position where successive series of observations are being made. This type of change may be termed a local sequence. It is frequently not possible to distinguish between these two important types of changes that may occur in the population of an area under investigation, though hydrographic data accompanying biological sampling will aid materially in the interpretation of the biological data by providing information on the nature of residual movements of water. Local sequences in populations are likely to be more sudden than individual population successions, since the latter depend upon biological development rather than upon a simple physical shift of water masses. The former may also at times be slow when neighboring populations become mixed or scattered only through the process of advection or lateral mixing.
An example embracing both local sequence and individual population succession in a population is illustrated by the recent observations of Redfield (1939) on the history of populations of the pteropod Limacina retroversa, its entrance and sojourn in the Gulf of Maine (see also p. 864). A population of small individuals appears in the Gulf in December with inflowing water from the east. Caught in the cyclonic circulation of the Gulf, they gradually decrease in number through mortality or some are
The changes occurring in a population may involve a succession of development stages of a given species. In view of this it should be noted that any biological succession observed may result from two causes. There may be (1) a change in composition of species, owing to different biological responses to physical or chemical changes (that is, rise or fall in temperature or nutrient state of the water) that have occurred within the individual water mass, or (2) a change in the relative maturity of the population, owing simply to the passage of time and to chronologically developed stages in the life history of the individuals of one or more species. A change in the phytoplankton involving a succession of species is well illustrated in boreal water, where in late spring or summer there is commonly a drop in the concentration of diatoms in a predominantly diatom plankton and an accompanying or following increase in dinoflagellates. Here two factors, in particular, are operative in the individual water mass: (1) an increase in temperature (owing to advance of the season), which favors the warmth-loving dinoflagellates, and (2) depletion of plant nutrients by the diatoms to a point suboptimal for their abundant proliferation, but still sufficient for the dinoflagellates, which are able to reduce the nutrients further and through their motility to adjust themselves in some degree with respect to favorable light conditions (see p. 765). The phosphate and nitrate requirements of certain dinoflagellates (Ceratium sp., Peridinium sp., Prorocentrum micans) have been found experimentally to be exceedingly low (Barker, 1935). The maximum rate of division has already been reached with 0.1 parts per million of nitrogen, and this element is probably a limiting factor only at dilutions of 0.01 to 0.001 parts per million.
A biological succession involving the percentage composition of developmental stages of a single dominant species associated with lapse of time is illustrated in studies of the life cycle of Calanus finmarchicus in the relatively slowly flushed waters of the Clyde Sea area (p. 323) where in Loch Striven it was possible (Marshall, Nicholls, and Orr, 1934) to trace the successive developmental stages and broods of Calanus that occur during the seasons.
Bibliography
Ardley, R. A. B., and N. A. Mackintosh. 1936.
“The royal research ship Discovery II”
. Discovery Repts., v. 13, p. 77–106, 1936.
Barker, H. A.1935.
“The culture and physiology of marine dinoflagellates”
. Archiv f. Mikrobiol., Bd. 6, 2 Heft, p. 157–181, 1935.
Bauer, L. A., W. J. Peters, J. P. Ault, J. A. Fleming1917.
“The magnetic work of the Carnegie, 1909–1916”
. Carnegie Inst. Washington, Pub. 175, Researches of the Dept. of Terrestrial Magnetism, v. 3, p. 157–165, 1917.
Birge, E. A., and Chancey Juday. 1922.
“The inland lakes of Wisconsin. The plankton. I. Its quantity and chemical composition”
. Wisc. Geol. & Nat. Hist. Survey, Bull. no. 64, Sci. Ser., no. 13, p. 1–222, 1922.
Bowditch, Nathaniel. 1934. Instruments and accessories in navigation. p. 11–40 in: American Practical Navigator, no. 9, Washington, D. C., 852 pp.1934.
Brooks, Charles F.1932. Oceanography and meteorology. Physics of the earth, v. 5, Oceanography, p. 457–519, Nat. Research Council, Bull.no. 85, 1932. Washington, D. C.
Buchanan-Wollaston, H. J.1925.
“A recording current meter”
. Conseil Perm.Internat. p. l’Explor. de la Mer, Pub. de Circonstance, no. 86, 14 pp., 1925.
Buchanan-Wollaston, H. J.1930.
“Note on a self-recording current meter”
. Conseil Perm. Internat. p. l’Explor. de la Mer, Rapp. et Proc.-Verb., v. 64, p. 33, 34, 1930.
Carruthers, J. N.1930.
“Further investigations upon the water movements in the English Channel”
. Marine Biol. Assn. U. K., Jour., v. 17, p. 241–275, 1930.
Defant, A.1929. Dynamische Ozeanographie. Naturwissensch. Monographien u. Lehrbücher, Bd. IX, Einführung in die Geophysik III, 222 pp., 1929. Berlin
Ekman, V. W.1905.
“Kurze Beschreibung einer Propellerstrommessers”
. Conseil Perm. Internat. p. l’Explor. de la Mer, Pub. de Circonstance, no. 24, 4 pp., 1905.
Ekman, V. W.1926.
“On a new repeating current-meter”
. Conseil Perm. Internat. p. l’Explor. de la Mer, Pub. de Circonstance, no. 91, 27 pp., 1926.
Ekman, V. W.1932.
“An improved type of current-meter”
. Conseil Perm. Internat. p. l’Explor. de la Mer, Jour. du Conseil, v. 7, p. 3–10, 1932.
Emery, K. O., and R. S. Dietz. 1941.
“Gravity coring instrument and mechanics of sediment coring”
. Geol. Soc. Amer., Bull.,, v. 52, p. 1685–1714, 1941.
Fulton, T. W.1897.
“The surface currents of the North Sea”
. Scottish Geogr. Mag., v. 13, p. 636–645, 1897. Edinburgh.
Gran, H. H.1932.
“Phytoplankton. Methods and problems”
. Conseil Perm. Internat. p. l’Explor. de la Mer, Jour. du Conseil, v. 7, no. 3, p. 343–358, 1932.
Hardy, A. C.1936.
“The ecological relations between the herring and the plankton investigated with the plankton indicator”
. Pt. I. The object, plan and methods of the investigation. Marine Biol. Assn. U.K., Jour., v. 21, p. 147–177, 1936.
Helland-Hansen, Björn. 1914.
“Eine Untersuchungsfahrt im Atlantischen Ozean mit dem Motorschiff Armauer Hansen im Sommer 1913”
. Internat. Rev. d. ges. Hydrobiol. u. Hydrogr., Bd. VII, p. 61–83, 1914.
Hough, Jack. 1939.
“Bottom-sampling apparatus”
. p. 631–664 in Recent Marine Sediments, A Symposium. Tulsa, Okla., A.A.P.G., 736 pp., 1939.
Idrac, P.1931.
“Le nouvel enregistrateur de courants sousmarins”
. Inst. Océanographique, Ann., v. 10, p. 99–116, 1931. Paris.
Iselin, C. O’D., II1933.
“Some phases of modern deep-sea oceanography”
. Smithsonian Rept. for 1932, p. 251–67, 1933.
Jacobsen, J. P.1909.
“Der Libellenstrommesser”
. Conseil Perm. Internat. p. l’Explor. de la Mer, Pub. de Circonstance, no. 51, 20 pp., 1909.
Künne, Cl.1933.
“Weitere Untersuchungen zum Vergleich der Fangfähigkeit verschiedener Modelle von vertikal fischenden Plankton-Netzen”
. Conseil Perm. Internat. p. l’Explor. de la Mer, Rapp. et Proc.-Verb., v. 83, 36 pp., 1933.
Marshall, S. M., A. G. Nicholls, and A. P. Orr. 1934.
“On the biology of Calanus finmarchicus. V. Seasonal distribution, size, weight, and chemical composition in Loch Striven in 1933, and their relation to the phytoplankton”
. Marine Biol. Assn. U.K., Jour., v. 19, no. 2, p. 793–828, 1934.
Moberg, E. G., and John Lyman. 1942.
“The E. W. Scripps”
. Scripps Inst. Oceanogr., Records of Observations, no. 1, p. 3–12, 1942.
Mosby, H.1940.
“An oceanographic thermo-sounder”
. Union Géod. et Géophys. Intern., Assn. d’Oceanographie Phys., Procés-Verb., no. 3, p. 190–91, 1940.
Murray, Sir John, and Johan Hjort1912. The depths of the ocean. Macmillan, London, 821 pp., 1912.
Nansen, Fridtjof. 1906.
“Methods for measuring direction and velocity of currents in the sea”
. Conseil Perm. Internat. p. l’Explor. de la Mer, Pub. de Circonstance, no. 34, 42 pp., 1906.
Parker, W. E.1932.
“Additional oceanographic instruments. Physics of the Earth, v. 5, Oceanography, p. 442–450”
. Nat. Res. Council, 581 pp., 1932.
Petersen, C. G. Joh. 1918.
“The sea bottom and its production of fish food. I. Apparatus for investigation of the sea bottom”
. Danish Biol. Station. Rept., no. 25, p. 1–6, 1918.
Pettersson, H.1915.
“A recording current meter for deep sea work”
. Roy. Met. Soc., London, Quart. Jour., v. 41, p. 65–69, 1915.
Pettersson, O.1913.
“Photographisch registrierender Tiefenstrommesser für Dauerbeobachtungen”
. Ur. Svenska Hydr. Biol. Komm. Skr., v. 5, 8 pp., 1913. Göteborg.
Piggot, Charles S.1936.
“Apparatus to secure core samples from the ocean bottom”
. Geol. Soc. Amer., Bull., v. 47, p. 675–984, 1936.
Pillsbury, John E.1891.
“The Gulf Stream. Methods of the investigation and results of the research”
. U. S. Coast and Geod. Surv., Rept. for 1880, Appendix no. 10, p. 461–620, 1891.
Pinke, F.1938. The expeditionary ship and the naval personnel's share. Snellius-Exped. in the eastern part of the Netherlands East-Indies 1929–1930. v. 1, p. 47–78, 1938.
Rauschelbach, H.1929.
“Beschreibung eines bifilar aufgehängten, an Bord elektrisch registrierenden Strommessers”
. Ann. d. Hydrogr. u. Mar. Meteor., Beiheft, 71 pp, 1929.
Redfield, A. C.1939.
“The history of a population of Limacina retroversa during its drift across the Gulf of Maine”
. Biol. Bull., v. 76, p. 26–47, 1939.
Revelle, R. R., and F. P. Shepard. 1942. Bottom currents at the California sea floor (to be submitted to Geol. Soc. Amer., Bull.)
Rudé, G. T.1928.
“Instructions for tide observations”
. U. S. Coast and Geod. Surv., Spec. Pub. no. 139, 78 pp., 1928.
Rudé, G. T.1938.
“New methods of marine surveying”
. Amer. Phil. Soc., Proc., v. 79, p. 9–25, 1938.
Schmidt, Johs. 1929.
“Introduction to the oceanographical reports. Danish Dana-Expeditions 1920–1922 in the North Atlantic and the Gulf of Panama”
. Oceanogr. Repts., no. 1, p. 1–20, 1929.
Seiwell, H. R.1929.
“Patterns for conical silk plankton-nets of one meter and half-meter diameters”
. Conseil Perm. Internat. p. 1’Explor. de la Mer, Jour. du Conseil, v. 4, p. 99–103, 1929.
Seiwell, H. R.1940.
“Anchoring ships on the high seas”
. U. S. Naval Inst., Proc., v. 66, p. 1733–1740, 1940.
Soule, Floyd M.1932.
“Oceanographic instruments and methods. Physics of the Earth, v. 5, Oceanography, p. 411–441”
. Nat. Research Council, Bull.no. 85, 581 pp., 1932.
Spiess, H. C. Fritz. 1932a.
“Das Forschungsschiff und seine Reise. Deutsche Atlantische Exped”
. Meteor 1925–1927, Wiss. Erg., Bd. 1, 442 pp., 1932.
Spiess, H. C. Fritz. 1932b.
“Mooring of ships in deep water for the direct measurement of currents”
. Hydrogr. Rev., v. 9, no. 1, p. 1–38, 1932.
Spilhaus, Athelstan F.1938.
“A bathythermograph”
. Jour. Marine Research, v. 1, p. 95–100, 1938.
Spilhaus, Athelstan F.1940.
“A detailed study of the surface layers of the ocean in the neighborhood of the Gulf Stream with the aid of rapid measuring hydrographic instruments”
. Jour. Marine Research, v. 3, p. 51–75, 1940.
Stetson, H. C.1937.
“Current measurements in the Georges Bank canyons”
. Nat. Research Council, Amer. Geophys. Union, Trans., p. 216–219, 1937.
Sverdrup, H. U.1929.
“The waters on the North Siberian Shelf”
. Norwegian North Polar Exped. with the Maud 1918–1925, Sci. Results, v. 4, no. 2, 131 + 75 pp., 1929. Bergen.
Sverdrup, H. U., and Odd Dahl. 1926.
“Two oceanographic current recorders designed and used on the Maud Expedition”
. Optical Soc. Amer., Jour., v. 12, p. 537–545, 1926.
Tait, J. B.1930.
“The water drift in the northern and middle area of the North Sea and in the Faeroe-Shetland Channel. Fishery Board for Scotland”
. Sci. Invest, no. 4, 56 pp., 1930. Edinburgh.
Thompson, Thomas G.1936.
“The motorship Catalyst—A seagoing laboratory”
. Jour. Chem. Education, v. 13, p. 203–209, 1936.
Thorade, Hermann. 1933. Methoden zum Studium der Meeresströmungen. Abderhalden's Handbuch der biologischen Arbeitsmethoden, Abt. II, Teil 3, p. 2865–3095, Berlin1933.
Tibby, R. B.1939.
“Report on returns of drift bottles released off southern California, 1937”
. Div. Fish and Game of Calif., Bur. Marine, Fisheries, Fish Bull.no. 55, 36 pp., 1939.
Uda, M.1935.
“The results of simultaneous oceanographical investigations in the North Pacific Ocean adjacent to Japan made in August, 1933”
. Japan, Imperial Fisheries Exper. Sta., Jour., no, 6, 130 pp., 1935.
Veatch,. A. C., and P. A. Smith. 1939.
“Atlantic submarine valleys of the United States and the Congo Submarine Valley”
. Geol. Soc. Amer., Spec. Paper, no. 7, 101 pp., 1939.
Winsor, C. P., and G. L. Clarke. 1940.
“A statistical study of variations in the catch of plankton nets”
. Jour. Marine Research, v. 3, p. 1–34, 1940.
Witting, R.1923.
“Om en till skeppsbord reporterande strömmätare”
. Festsschrift f. Otto Pettersson, p. 90–96, 1923. Helsingfors.
Wüst, Georg. 1932.
“Programm, Ausrüstung, Methoden der Serienmessungen”
. Deutsche Atlantische Exped. Meteor 1925–1927, Wiss. Erg., Bd. IV, 1 Teil, p. 1–59, 1932.
Wüst, Georg. 1933.
“Thermometric measurement of depth”
. Hydrogr. Rev., v. 10, no. 2, p. 28–49, 1933.
ZoBell, Claude E.1941.
“Studies on marine bacteria. I. The cultural requirements of the heterotrophic aerobes”
. Jour. Marine Research, v. 4, p. 42–75, 1941.