SCIENCE, THE OPEN DOOR
7—
Iowa State—
The Young Professor
The class was over. I had concluded a discussion, with various examples, about electricity—about electromotive force and voltage, current, electrical resistance, and power. A young student came up and said, rather shyly: "I think I understand what you were saying, but there is one point I don't understand. Why do you need two wires to an electric light? Doesn't the electricity just flow in and get used up?"
Obviously, Iowa State was not MIT. I realized my discussion needed refinement.
Although my formal education had ended, Iowa State was providing other invaluable, on-the-job learning experiences. I learned how to teach in small classes and large, undergraduate and graduate; how to adapt to the mores of a large, public, state-supported university; how to establish and fund one's own research laboratory and program; how to advise graduate students, each with a unique background and personality; how to live in a small Iowa community.
Iowa State College (now University) of Agriculture and Mechanic Arts was one of the land-grant colleges established under the Morrill Act of 1862 to provide "practical" education. The more traditional liberal arts curricula and the professional schools of law and medicine were at the University of Iowa in Iowa City. Agriculture and engineering were the prominent programs at Ames, supported by a division of industrial science (mathematics, physics, chemistry, and biology) and accompanied by veterinary, medicine and home economics. (Female students comprised about 25 percent of the undergraduates, mostly in the
home economics program.) There were also supporting programs in the humanities, social sciences, and arts. Graduate programs were well established in agriculture, engineering, and the basic sciences.
The rather handsome campus had been designed for some six thousand students. Now, with the flood of returning veterans, enrollment had exceeded twelve thousand and was, in 1949, still at eleven thousand. Trailers for offices and classrooms dotted the campus and laboratories, and lecture rooms were used evenings, Saturdays, and even Sundays to provide all of the needed classes.
The returned veterans were older, more mature, more serious. They knew why they were at college.
Iowa State was in the top rank of the second tier of research universities in the U.S., except in agriculture, for which it was one of the very best. Its faculty included several members of the National Academy of Sciences and had high standards. Except for the agriculture department, which had considerable federal and state research support, the campus was limited by the available resources. The new Atomic Energy Laboratory at Ames was permitting a considerable expansion of the faculty and research activity in selected areas of physics and chemistry, but a broader lack of funds severely restricted the campus's ability to provide basic research equipment or to "ante up" matching funds for grants and imposed relatively large teaching duties. In consequence, the campus sought to recruit able young Ph.D.'s, nurture and exploit their bright ideas and enthusiasm "on their way up," and then reluctantly let them go, knowing it could not in general match the opportunities available at the leading research institutions.
Physics departments have a large "service" load, providing instruction in basic physics to students in many disciplines. All of the faculty, senior and junior, in the physics department at Iowa State participated in the teaching of elementary physics. It is a nice tradition, emulated in many physics departments, and one which I think would be salutary, in other sciences such as biology, or chemists. This practice keeps all of the faculty in touch with the basics of their science and with the always changing flow of incoming students.
At Iowa State four different elementary, physics courses were taught. The largest, of over one thousand students, was for engineers. Other, distinct courses were offered for science majors, for veterinary medicine students, and for home economics students. The physics faculty, at that period was almost bipartite, split between the older professors, trained in classical physics before 1930, and the younger members, able and
eager to apply quantum mechanical ideas to a variety, of physical science questions. The former, now largely excluded from modern research, assumed primary responsibility for organizing the large service courses.
In addition to launching a research program with graduate students, I taught a graduate course in biophysics and two sections of one or another of the basic physics courses throughout the academic year. Although I had taken more than three years of physics courses at MIT, I quickly realized the truism that "you really learn a subject when you teach it." In order to teach a subject to others, one must thoroughly understand it oneself and must be able to adapt one's presentation to the initial level of comprehension of the students. Their questions can force unexpected insights. I acquired a much deeper grasp of basic physics through that experience.
Teaching sections of physics for the engineering students provided a special opportunity to appreciate the logistics of the provision of mass education. With nearly a thousand students and no lecture hall that seated more than 350, the demonstration lectures had to be repeated three times. I sat in on one of these to know what had been presented. With twenty-five students in each recitation session, there were forty. such sessions in which homework assignments were returned and problems worked out, questions answered, and, if time permitted, further illustrations of physics presented. The instructors of all the sections met weekly to ensure coordination.
Two mid-term examinations and a final examination were given. In the days before machine grading, one instructor—sometimes me—graded one problem on a thousand examinations. There was inevitably some rivalry among instructors as to how well their sections would do on the examinations, but of course the statistical variations of student quality, overwhelmed pedagogical ability. At the end of the quarter, all of the scores from examinations and homework assignments were summed and letter grades assigned. Since the cut-off points were arbitrary, a score of 891 might be an A and 890 a B.
Such mass education is economical, but it has many flaws.
This course provided my first contact with one of the corrupting influences in American higher education, big league athletics. One day I received a call from the football coach. A particular student in my physics section, who was doing poorly, was very important to the football team. Could I see my way to a more lenient grade so that he would be eligible to play next year? I thought quickly and responded that such actions were not my policy, but that I could recommend some physics
graduate students who would be pleased to make some money by tutoring this student. This proved acceptable and the student's work actually improved significantly. I learned subsequently that such calls were not uncommon.
The course in biophysics, four lectures per week for thirty weeks, was a challenge. There was no text available. I attempted to analyze and present the physical principles underlying biophysical instrumentation (e.g., optical microscopes, the electron microscope, spectrophotometry, light scattering, X-ray diffraction, measurement of radioactivity, and so on), to discuss the biological effects of radiation (electromagnetic and ionizing), and to consider the physical properties of living tissue (primarily electrical). I had much to learn and I found my training in mathematics was often the essential key. The first year I averaged ten hours of preparation for each hour of lecture.
As might be expected, the undergraduates at Iowa State came mostly from the towns and farms of Iowa. In contrast to a highly selective private school like MIT, Iowa State admitted nearly every high school graduate in Iowa who applied. But then many could not achieve to the college's standards, and the freshman attrition rate g as high.
Graduate students were drawn more broadly, although primarily from the Midwest. The college was obliged to accept a rather high proportion of graduates from the state's many four-year colleges, resulting again in a high first-year attrition (about one third of the incoming graduate students in physics dropped out). Only a few of the physics graduate students and some biochemistry majors (from the chemistry department) were interested in biophysics. Biology at Iowa State was unfortunately fragmented pragmatically into several divisions: zoology, botany, microbiology, and genetics. Other subdivisions of economic importance (e.g., entomology.) were located in agriculture. For most of the biology faculty, biochemistry and biophysics were arcane subjects, and their undergraduate curricula required neither calculus nor physics.
I felt strongly that the lack of such requirements was a grievous deficiency that would handicap the biology, students throughout their future careers. But, despite repeated efforts over the years, I was unable to effect a change.
Agriculture was the primary locus of biological research funding on the campus. I was fully confident that research on nucleic acids, such as I was undertaking, would be key to the long-range future of agriculture, and I attempted on several occasions to persuade the dean of agriculture of this view. He listened attentively but was never persuaded. In truth,
agricultural research of the conventional kind was doing very well in that period and agricultural yields were increasing yearly. Twenty-five years before recombinant DNA, my enthusiasm, albeit justified, was, pragmatically, premature.
Iowa in 1949 was primarily a rural state with no large city and was composed mostly of farms and small, relatively isolated communities. It was little changed socially from 1910 or 1920. Alcohol could be purchased only at state liquor stores in the county seat. The sale of margarine was illegal. Many major issues of the time (the Rosenberg trial, the Hiss affair) penetrated little into our consciousness.
Life in Ames had an even, pleasant tenor. It was a small community, a university town, with seven to eight thousand permanent residents and eleven thousand students. Ames was small-town America, lacking the extremes and diversity of a city—there was no great wealth and no real poverty. Crime was almost nonexistent and doors were seldom locked. When I arrived, there were no stoplights, no large stores, few (and mediocre) restaurants. There were no "minority problems." There was a genuine small-town kindliness and neighborliness. As might be expected in a university town, the public school system was excellent. It was an ideal place to raise children.
There was almost no rental housing in Ames. All of the permanent residents lived in their own houses. Most of the students were housed on campus, the women's dormitories on the east side, the men's on the west. To accommodate the influx of married students, a sea of Quonset huts named Pammel Court had been erected—each hut was made into a small two-bedroom house. We lived there for our first two months. Having just completed graduate study, I could relate to the concerns of the surrounding students, even though I now had a different status.
We then located a frame house, about thirty years old, four blocks from the college on a dead-end street. The house backed onto a college experimental farm and was two blocks from elementary school. My father loaned me two thousand dollars for the down payment, and a 2.5 percent FHA mortgage of sixty-five hundred dollars covered the rest. My annual salary was fifty-six hundred dollars, out of which four hundred dollars was deducted for the retirement plan. The remainder was enough to get by on in Ames in 1949.
The cultural life of a small Iowa community can be quite minimal, but in Ames the presence of the college provided a wealth of activities. In addition to the plays and concerts that the campus itself generated, there was a visiting musical artist series, including symphony orchestras,
and there were guest lecture and foreign film series. And of course there were the athletic contests, especially football and basketball, and a great student-organized fall harvest/football homecoming festival, VEISHEA (Veterinary medicine, Engineering, Industrial Science, Home Economics, Agriculture), with a parade of fanciful floats.
Television was just then making its first incursions into this semirural idyll. We acquired our first TV set, a small black-and-white model. We had resisted this "invasion" of the outer world for years, but we found that the children were disappearing to the neighbor's houses to watch. Also, references to TV programs of which our children were ignorant were made in school classrooms. TV had already become an integral part of the culture. To my great surprise, I was, initially, mesmerized. To be able to watch movies in one's own home had been an undreamed of luxury, available only to movie magnates and presidents. Now we had this privilege! Of course, the fascination wore off, and after a time I could restrict TV to a reasonable share of my existence. Indeed, familiarity breeds contempt. As I became accustomed to this technological marvel, I became increasingly selective and guarding of my time. Today with cable we have thirty-six channels—and often nothing I want to watch.
Iowa State provided my first introduction to campus politics—the intricate network of relationships and personalities that parallels, and sometimes displaces, merit in the allocation of resources and rewards. Iowa State had conventional hierarchical lines of authority. Mine ran through the department chairman to, variously, the dean of science or the director of the Ames Laboratory of the AEC (which provided much of the research funds available to physics) and (of limited access) through the dean to the president. The Ames Laboratory had no man-date for biophysical research. Thus, while I was able to "bootleg" shop time and instrument repair from their well-maintained facilities, I received no direct support from that source.
The dean had no budgetary item for research equipment. Iowa operated on a biennial budget If, as the end of the biennium approached, unexpended funds remained in the college budget, the dean received a sum that he could use to meet the numerous requests as he believed best. He was clearly influenced in this regard by his personal relationships with the department chairmen. It was also widely recognized that he hoped to be the next college president and his allocations reflected judicious efforts to strengthen his political base, at least on the campus. (He did not succeed.)
It soon became very clear that, if a faculty member had a personality clash with his department chairman—who was appointed by and was often a friend of the dean—his situation on the campus was hopeless. Regardless of academic merit, he could be denied resources, limited in laboratory space, assigned excessive teaching responsibilities, passed over for promotion, denied increase of salary, and so on. And this happened, painfully, to some. In one especially grievous instance, I attempted to intervene at the dean's level and was somewhat curtly rebuffed.
Fortunately, my relations with my department chairman were excellent. And indeed, while some of the physics faculty probably were perplexed as to the significance of biophysics, in general my relations with the other faculty were warm, and close friendships developed between families. Of course, it may have helped that that department was rather well supported and I was not a competitor for their funds.
As a public institution, Iowa State was subject to a variety of regulations and policies more appropriate to a department of highways or a water commission than to an institution of higher learning. Purchasing, accounting, and time-reporting procedures were rigid and occasionally stultifying. Indeed, there was at times a sense that we were public servants, "hired hands," available at the public's call to perform various functions to their satisfaction.
A tragedy that occurred in my first year at Iowa State has left a sharp, poignant memory. Frank Carlson, a theoretical physicist and a student of Robert Oppenheimer's, had been with me at the Radiation Laboratory and was now on the faculty at Ames. A thoughtful, sensitive, private man, we quickly became good friends. One morning, I saw him standing in the hallway outside the physics department office with a dazed, distressed look as swarms of students swirled by him, to and from their classes. He was motionless and I thought to push through to him to ask if he were all right, but I had to hasten to my class and thought I'd speak to him later. I tried to find him later that day, but he was not in his office. Next morning, we learned that Frank had hanged himself in his basement. He had been increasingly depressed for some time; psychiatric help had been of no avail.
In our small world, this was a searing shock—and I felt, and still feel, a deep remorse that I had not followed my instinct to reach him. Since, I have always responded at once to a look of distress. There may not be a second chance.
It soon became evident that I would have to find an outside source
of funds for my research. The department provided some initial "startup" equipment, but money for supplies, chemicals, a laboratory assistant, and so on would have to come externally. I intended to pursue my research into the nature of the effects of ultraviolet radiation on the nucleic acids. I knew the Rockefeller Foundation had been interested in John Loofbourow's ideas at MIT so I applied to them. With, I suspect, Loofbourow's recommendation, I received a grant of five thousand dollars per year for two years. I could get underway!
Toward the end of my first year at Iowa State, I saw a notice that a Gordon Conference—an intense, week-long gathering of experts—was to be held that summer on the subject of nucleic acids and proteins at a small preparatory school in New England. This seemed a fine opportunity to catch up on the most recent findings in the field. Jesse Scott and I both applied to attend and were accepted. This meeting would also provide my first opportunity since leaving MIT to visit with John Loofbourow in Cambridge on my way to the meeting.
A few weeks before the meeting, I received a shocking telegram. John Loofbourow had died suddenly of a massive heart attack. I felt a deep and continuing loss. John had been my mentor, guide, scientific role model, friend. We would never again share happy hours of discussion and scientific inspiration. I am sure this early and tragic loss of my mentor significantly influenced my subsequent career. I know there have been numerous times I would have liked his advice, his calm wisdom. And of course, in the network of science his backing could have provided forms of access that I subsequently lacked.
John's wife, Dorothea, was grief-stricken and, one week later, she too died of a heart attack. A stunning blow.
The Gordon Conference went on as planned. It was a remarkable event. There were some ninety-five participants who included almost everyone who was doing physicochemical or structural or metabolic studies on the nucleic acid or protein molecules—Paul Doty, John Kendrew, and many others. Since 1950, this field has expanded again and again. For simple reasons of size, the researchers of nucleic acids and proteins had to divide into separate conferences by the early 1960s, and the nucleic acid sessions soon began to alternate, as between structural studies and metabolic and functional studies. Today only a small sector of each field can be discussed at any one conference.
8—
Iowa State—
Research and Discovery
In 1949, much of the physics and chemistry of life was still utterly mysterious. Ill-defined, large molecules called proteins apparently catalyzed most essential chemical reactions and provided structural and contractile components. The origin—the manner of synthesis—of these proteins was completely obscure.
Several lines of evidence implicated the nucleic acids in genetic processes and suggested that they might indeed convey genetic information. If so, they had to play a primary role in protein synthesis. But knowledge of the structures of the nucleic acids was even more tenuous than that of proteins. We knew that there were two broad classes: deoxyribonucleic acid (DNA) and ribonucleic acid (RNA). Both were chains of monomers, nucleotides. They differed chemically in the sugar portion of each component nucleotide, deoxyribose in DNA, ribose in RNA. In addition to common nucleotides, each contained a distinctive nucleotide, thymidylic acid in DNA, uridylic acid in RNA. All cells contained both DNA and RNA. Some viruses contained DNA, some RNA.
In contrast to the proteins, some of which had even been crystallized, no one had ever obtained a pure, single DNA or RNA species or indeed, as far as was known, a complete "molecule." Only complex mixtures, mostly fragments, were available. And, if a pure, single species had been available, there were no means to analyze its composition at the nucleotide level or to characterize its functional role. Nothing was known about the synthesis of nucleic acids or of their metabolic turnover, if any. Yet these now appeared to be the carriers of inheritance.
In science, the choice of a specific field of research and a means of approach is critical. When much is unknown, it is very difficult to forecast which approaches or paths will be fruitful and which will be dead-ends. My choice—to explore the domain of the nucleic acids and to seek to elucidate as much as I could of their structures and, in time, their functions—was most fortunate. The nucleic acids, which had occupied a minor and obscure niche in biochemistry, were about to move to the center of the biological stage for the next several decades. So little was known about the nucleic acids that almost any new insight would be valuable. And, in 1949, there were few competitors in this biochemical backwater.
With my background at the Radiation Laboratory and in spectrophotometry, and located in a physics department with access to (then) modern spectrophotometric equipment (for infrared and Raman spectroscopy), and provided with graduate students who had backgrounds in physical science and were familiar with physical instrumentation, it was natural for me to seek to explore the structure of nucleic acids by means of their interaction, in various modes, with electromagnetic radiation—to study the absorption, re-emission, and scattering of infrared, visible, and ultraviolet radiation by nucleic acids and to look for photochemical changes induced by such interaction. Studies of this nature had been very powerful means of elucidating the structures of simpler organic molecules and it seemed reasonable to believe they would provide a valuable approach to the study of nucleic acid structures.
We knew that DNA molecules were long, polymeric chains of deoxyribonucleotides. Four different subunits were known—adenylic, guanylic, thymidylic, and cytidylic. Each was composed of a complex ring structure, either a purine (adenine or guanine) or a pyrimidine (thymine or cytosine), joined to a sugar (always deoxyribose), joined in turn to a phosphate group. The subunits were linked together through the phosphate groups between the sugars. The purines and pyrimidines were strong and distinctive absorbers of ultraviolet light.
For the research I wanted to undertake at first, it seemed preferable to study the true nucleic acid subunits, the deoxynucleotides, rather than the isolated purines and pyrimidines. However, in order to do this, I needed a source of deoxyribonucleotides. They were not commercially available then and for good reason—the best available methods for their isolation provided yields of less than 1 percent from a DNA source. Nor was an undegraded DNA of good quality then commercially available.
One of the major heuristic differences between the biology of the
1950s and the biology of the 1990s is the presence today of numerous small companies that supply, for a price, a wide variety of the chemicals, enzymes, even viruses and cells employed in biological research. This development, largely concentrated in the United States, has greatly accelerated the pace of biological investigation. In the 1950s, one had to prepare one's own DNA, as well as the various enzymes needed to digest or modify it, starting usually from fresh animal tissue obtained from a slaughterhouse. Each preparation was not only time-consuming but often involved an interim research project to define the optimum conditions for each step or to apply new techniques that hopefully would provide more acceptable yields.
By preparing our own DNA, purifying our own enzymes, and introducing new fractionation methods, we were able for the first time to digest DNA completely to its component mononucleotides and to prepare these quantitatively. In addition to the four principal mononucleotides we expected (adenylic, thymidylic, guanylic, and cytidylic), we found a minor fifth mononucleotide in our digest that proved to contain the then recently discovered pyrimidinc, 5-methylcytosine (a modified form of cytosine).
The mononucleotides were then used for the planned ultraviolet irradiation studies, as well as for other research such as pioneering Raman (the study of the spectra of re-emitted radiation) and infrared absorption spectroscopy. This information could establish the tautomeric state (i.e., which of several possible alternative atomic groupings was predominant) of the various substituent groups on the purines and pyrimidines. The ultraviolet irradiation studies demonstrated that, on exposure to ultraviolet, the cytidylic acid underwent a reversible loss of its ultraviolet absorption similar to that we had previously described for uracil and uridylic acid but more rapidly revertant. The other deoxynucleotides were more resistant to ultraviolet irradiation.
The infrared absorption studies were performed with solutions of the nucleotides. These experiments were complicated by the fact that water itself has strong absorption bands in regions of the infrared spectrum. To circumvent this difficulty, we also studied the infrared absorption of the nucleotides dissolved in heavy water (D2 O), as its absorption bands are shifted relative to those of H2 O. These studies permitted the assignment of many absorption bands to specific atomic groups in the deoxynucleotide molecules and established that, under biological conditions, they are in what are known as the keto and amino configurations, as opposed to the alternative enol and imino configurations.
These chemical distinctions are very important in determining the kinds of secondary bonds that can then be formed between the nucleotides in higher level structures. The determination of these structures was confirmed by the results of the Raman spectroscopy. In those days, before lasers, the latter experiments were very difficult to perform because of the low light levels available.
It was known that some viruses, such as the tobacco mosaic virus, did not contain DNA but had the other form of nucleic acid, ribonucleic acid, RNA, as their (probable) genetic material. I therefore wanted to study RNA. RNA is abundant in cells, but at that time it could only be isolated as a complex mixture. To obtain a pure, individual RNA, I therefore undertook to grow and isolate the tobacco mosaic virus. After all, Iowa State was an "Ag school" and as such had well-equipped greenhouses. The authorities were at first unenthused about my growing stocks of infected tobacco plants in their greenhouses (the virus can also infect such plants as tomato), but reluctantly they consented.
The tobacco mosaic virus had been isolated and purified to a quasi-crystalline state by Wendell Stanley in the 1930s. The dimensions of the virus particle had been determined in the electron microscope. It had a particle mass of approximately forty million daltons. By chemical analysis, it was known to contain about 5 percent RNA. However, it was quite unknown whether there was one RNA molecule (of mass two million daltons) in each particle or a set of several smaller molecules, which might or might not be identical. We sought to resolve this question by performing light-scattering studies on the isolated viral RNA. We built and calibrated our own light-scattering apparatus. By measurement of the light scattered at various angles from a solution of macromolecules, one can determine the number of scattering particles and therefore, knowing the concentration of the solution, their molecular mass. One can also, assuming a structural model, determine their spatial dimensions.
We introduced a new concept. By measuring the scattering at different wavelengths in the visible and ultraviolet, we were able to differentiate between various possible structural models to determine, unambiguously, the spatial dimensions of the RNA. Our results demonstrated that, when carefully prepared, the RNA was a single molecule of mass two million daltons.
We were, step by step, establishing solid facts about the nucleic acids and their components: absolute absorption coefficients, confirmed
structures, molecular masses and dimensions. We needed, however, a more direct link to function.
In the 1940s, microbiological assay and chromatographic methods are developed that permit the quantitative determination of the amino acid composition of proteins. Arthur Martin, Richard Synge, Wilham Stein, and Stanford Moore play leading roles in this development Building upon these methods, Fred Sanger develops techniques for the determination of amino acid sequence within peptides and over the period 1951 to 1955 determines the first complete ammo acid sequence of a protein, insulin. With this advance, proteins, with all their critical biological functions, can be regarded as complex but precisely definable organic molecules.
In 1950, Linus Pauling and Robert Corey propose the alpha-hehx and the beta-pleated sheet models for the spatial arrangement of ammo acids in proteins. These structures, since verified as components of numerous proteins, provided the first valid concepts of the spatial organization of proteins.
In 1952, Alfred Hershey and Martha Chase demonstrate that DNA is (most likely) the genetic component of the bacteriophage T2, i.e., that DNA (without protein) can be the exclusive carrier of genetic reformation.
9—
Max Delbrück—
A Caltech Interlude
For an audience of generally reserved physicists, the applause was extraordinary. Max Delbrück had just concluded his third lecture on his path-breaking research with bacteriophage. These studies, over a fifteen year period, had brilliantly transformed a microbiological oddity into a principal means for the advancement of molecular biology, genetics, and biophysics.
The lectures were stirring. The research, the clarity of its conception, the elegance of its execution and interpretation, and the lucidity and sophistication of its presentation caught everyone's imagination and enthusiasm. Biology, a messy and descriptive field to most physicists, could be approached in a quantitative, analytical manner often using mathematical insight to produce clear, unambiguous results.
Frank Spedding, the director of the Ames AEC Laboratory, had made funds available to invite distinguished visiting lecturers. My nomination of Delbrück had been approved, and to my great delight he had accepted the invitation to stop over on his way to some East Coast meetings. We had never met. Delbruck was tall, thin, and crew cut with an energy that belied his forty-five years. In his three days, he met with most of the more prominent research faculty and, I believe, was pleasantly surprised by the activity he found on his first visit to a midwestern "Ag school."
I had become increasingly convinced that my biophysical and biochemical studies on nucleic acids would be much more meaningful if they could be related to a functional biological process. The long-range
goal was to understand what nucleic acids did, how they did it, and how their activity was controlled. The bacteriophage system as developed by Delbrück seemed ideal for my purpose. After his visit in the fall of 1951, we remained in contact. When reformed of my interest, he proposed that I should come to his laboratory at Caltech to learn the current state of bacteriophage biology and associated techniques.
In my third year at Ames, I was not yet eligible for sabbatical leave. However, I could take six months unpaid leave. I sought fellowship support for the six months. After my interview, I was informed by the fellowship board that they would award me a fellowship not for six months, which they thought would provide inadequate training, but for one year! My dilemma was happily resolved when Max was able to offer me a six-month fellowship from Caltech itself. I was to start on 1 January 1953.
We left Ames before Christmas in the midst of a sleet storm, wended our way across the frozen Midwest to Texas and on to Arizona, and spent Christmas in the snow at the Grand Canyon. Finally, we crossed the Mojave and descended through Cajon Pass into southern California. Once again, I had the sudden shock of emerging from bitter winter into warm sunshine and palm trees and fragrant flowers. We were just in time for our first Rose Parade.
Caltech was a revelation. Here were "giants"—scientists of the highest eminence—in almost every field. Lee DuBridge, who had directed the Radiation Laboratory, had assumed the presidency of Caltech in 1946 and had revitalized the institute. He had brought George Beadle, the leading geneticist, to head biology, and along with him Norman Horowitz and Herschel Mitchell. Alfred Sturtevant and Ed Lewis continued Thomas Hunt Morgan's drosophila research. Frits Went and James Bonner led an outstanding program in plant physiology. And Max Delbrück, Renato Dulbecco, and Jean Weigle were preeminent in bacteriophage research.
In chemistry, Linus Pauling was preeminent in the field of molecular structure, aided by Bob Corey. Jerry Vinograd, Walter Schroeder, and Norman Davidson were other leading chemists in the Pauling orbit. Jack Roberts had recently arrived to launch his NMR (nuclear magnetic resonance) program. In physics there were Richard Feynman, Murray Gell-Mann, Carl Anderson, and Charles Lauritsen; in geology Charles Richter and Benno Gutenberg; in astronomy Jesse Greenstein, Horace Babcock, and Martin Schmidt; in engineering Clark Millikan, Hans Liepman, and so on.
In a large university, a "post-doc" in Delbrück's laboratory such as myself might never have even seen these great men. But Caltech is a small, surprisingly democratic institution, and I met all of them and had significant discussions with several. Caltech was clearly a world center for science, one of the leading two or three in the world. In biology, almost everyone of distinction visited and lectured periodically. The newest discoveries were quickly known and discussed in a ferment of ideas. Biology at MIT had been much less dynamic, and the contrast with the near-isolation of Ames was extreme.
Max Delbrück was a profound influence on many students and colleagues, largely through the sheer force of his personality. Bright and rigorously logical, he imposed a quantitative intellectual discipline on the field of bacterial virology, which had been largely qualitative and unfocused. As the founder of a revived bacteriophage research program, Delbrück served as a combination pater familias and Herr Professor for the informal college of phage researchers developing about the country. He had insisted that they exchange cultures and limit the number of different bacteriophages studied so that different laboratories could obtain comparable results. Any new findings were quickly communicated to Delbrück for his comment and evaluation and through him to the community. He had a remarkable status and for a while produced an extraordinary cohesion within the phage fraternity. Of course, in time the field outgrew its founder.
In scientific debate, he could be mercilessly caustic but never mean-spirited. Delbrück had a weekly phage seminar at which ongoing research was discussed, or a visitor lectured. Max was known for his blunt and open criticism, his insistence on clear concepts and logical presentation, and his persistence in exposing ambiguities and uncertainties in a speaker's chain of reasoning. Many a speaker in his seminar or in the general biology seminar was sharply deflated; however, the net effect was to set and enforce a high standard of presentation.
His approach with students was generally "sink or swim"; it was salutary for the more resourceful, devastating for others. At the same time, Max was fond of playing the father-professor role, throwing large and often elaborate parties for the phage group at his house near campus, and taking caravans out for camping trips on the desert. The desert was quite unpopulated in those days and even a large group could be quite isolated in its own valley. These excursions featured long hikes and climbs and explorations of caves. At night, the brilliance of the stars
in the clear air, absent city lights, was startling to one raised in the always slightly hazy Midwest and East. Lying out in the open and watching the heavens revolve enabled me to comprehend how significant and mysterious the nightly stars must have seemed to primitive man.
Max had several "Maxims." For delivering a lecture to an audience of uncertain background knowledge, his advice was to "assume they are totally ignorant and infinitely intelligent." When skeptical of a new result, he would say, "I don't believe a word of it."
Max was convinced that important principles should be explicable in a simple and straightforward manner. If a convoluted and arcane argument was used, he was dubious His comment "what a swindle" indicated his belief that some big step had been overlooked or some error had been made in the complex exposition. He was frequently bemused by the progressivity of evolution—that each evolutionary advance, presumably achieved by selection to cope with a particular environmental circumstance, seemed again and again to bring additional new and unexpected potentials. "Nature provided more than was needed." The T4 bacteriophage he had studied proved to have over 150 genes, many of which would be of significant utility only in quite exceptional circumstances. The evolutionary steps that converted our anthropoid ancestors into Homo sapiens could hardly have been intended to solve problems in X-ray diffraction or quantum mechanics.
Delbrück's own research had mixed success. A physicist by training and inclination, he had little taste for the complexities and variations of chemistry. He sought out problems that could be approached quantitatively, analyzed abstractly, and preferably studied with simple equipment. This bent succeeded admirably in his early studies of bacteriophage, in the quantitative analysis of mutations and the discovery and analysis of bacteriophage genetics, and in his insistence that workers in bacterial virology should focus their efforts on a limited set of viruses.
But this approach was much less successful in his later studies of the biological effects of ultraviolet radiation on bacteriophage—in retrospect, the analysis was hopelessly complicated by the existence of multiple cellular repair mechanisms—and in his prolonged effort over twenty years to establish the mold Phycomyces as a model system for the study of sensory processes. This last was doomed by the inability to develop Phycomyces genetics. At the symposium in celebration of fifty years of biology at Caltech, Max, as chairman of a session, gently chided Dale Kaiser for having spent too many years researching the lambda
bacteriophage, long after the more significant discoveries had been made. Dick Feynman, sitting next to me, leaned over to say that Max should have applied his advice to his own work on Phycomyces .
As a physicist, Max sought to find principles of great generality, as opposed to solutions involving specific chemical interactions. It was his initial hope that the phenomena of genetics—cloaked in obscurity in the 1930s—would prove to rely on principles of physics previously undiscovered. In this, of course, he was grievously disappointed.
Much later, one of the most pleasant activities of my tenure as chairman of biology at Caltech was to organize the celebration for Max after he received the Nobel Prize in 1968. Admired, even exalted, for two decades at the institute, Max was felt by all there to deserve the Nobel Prize for his bacteriophage work. But after he had been passed over for so many years, and biology had moved on, many feared it was not to be. This made for all the more delight when the word came.
Max took the ensuing hoopla with his usual diffidence. I well recall the news conference the next day, with Max attempting to display and explain his current work with Phycomyces, which was completely unrelated to the research for which the prize was awarded. Prominent among the newspeople was a TV reporter who did a nightly interview with a local personality. The evening before, she had interviewed the superintendent for sewers. (Science reporting was at a low ebb in those days.) Actually, while Max was very pleased at the belated recognition, he was a little embarrassed that it had come long after he had left the phage field. He could no longer cogently discuss the current state of the science; bacteriophage research had passed him by. Knowing this, he would deprecatorily call himself "the old windbag" at the inevitable required speeches.
The celebration parts took the form of an elaborate musical skit with various members of the biology division commemorating and commenting in humorous doggerel on the several phases of Max's career. It was a loving "toast and roast" of a deservedly admired and venerated presence who had finally been appropriately honored.
At Caltech, I was primarily a student. I learned phage techniques by working through a lengthy set of prescribed laboratory exercises—I recall Max watching amusedly as I first learned to master sterile technique, that is, the art of holding all the tubes and cotton plugs while transferring liquid from one container to another. I also learned the current state of phage research in the group discussions. But I learned far more of biology and chemistry by attendance at the great variety of seminars
and from conversations with visitors and Caltech faculty and researchers, almost osmotically absorbing the ambience. Those six months introduced me to science at a still higher level of intensity and synergistic interaction.
In 1953, James Watson and Francis Crick propose the double helix structure of DNA, based upon the X-ray diffraction studies of Maurice Wilkins and Rosalind Franklin This structure made explicable in chemical terms many of the previously mysterious properties of genes and opened the way to molecular genetics.
10—
A Sidelight on Watson and Crick:
(A Corollary to Murphy's Law: Seekers/Finders >> 1)
The elucidation of the double helix structure of DNA by Watson and Crick has taken on mythic dimensions. The authors of the myths, non-scientists and (even) scientists like Watson himself, at the time ignorant of the history of DNA research, have presented a sometimes self-serving scenario akin to the primitive myths of creation in which the world—or the DNA structure—is derived from a formless void. Of course, it wasn't like that.
Science today is a cumulative enterprise rising step by step on a staircase of four centuries of sustained investigation and analysis. Each step has a background and a context. Indeed, the quick acceptance of the double helix structure derived from the fact that it made coherent and even plausible so many prior observations from genetics and DNA biochemistry.
As a participant in DNA research throughout this period, I had a close-up and somewhat bemused view of the ongoing research. As is the norm in science, several lines of research, including my own, were converging on the structure of DNA. The concept then of the double helix was, in itself, not so large a step—but it was a step that brought biology onto a new plateau with wide horizons and numerous paths to explore.
By the early 1950s, several laboratories were intensively engaged in the study of DNA. Arthur Kornberg's laboratory, then at Washington University and later at Stanford, sought to unravel the biochemistry of
DNA synthesis. Paul Doty's laboratory at Harvard applied powerful tools to the analysis of the peculiar physical chemistry of DNA. Alfred Mirsky at Rockefeller Institute had measured the DNA content of cell nuclei of varied species while Erwin Chargaff had shown that the purine and pyrimidine composition of these DNAs always displayed a curious regularity: the moles of adenine equaled those of thymine and the moles of guanine equaled those of cytosine. In London, Franklin and Wilkins sought to improve on the earlier X-ray diffraction studies of DNA by William T. Astbury.
I too, by a somewhat circuitous route, had become interested in aspects of DNA structure and sequence. In the course of my earlier studies, intended initially solely to provide the mononucleotides I needed for irradiation studies, I realized I had also opened an avenue into questions of nucleotide sequence and DNA structure. For in the course of the mononucleotide preparation, we at one stage reduced the DNA to a mixture of small polymers of an average length of four nucleotides. If we could fractionate this mixture we would be in a wholly unexplored realm, since until then no one had ever isolated, identified, or purified oligonucleotides (short strings of nucleotides) of any size or variety.
We found that we could indeed separate the digest into dinucleotides, trinucleotides, and larger fractions. And further, by careful choice of conditions, we could fractionate all of the dinucleotides according to their particular nucleotide composition. The ultraviolet absorption of each dinucleotide suggested its composition. This could be verified by degrading each to its mononucleotides and fractionating these.
This work proceeded well and brought us, for the first time, a small step into the world of DNA sequence—the order of nucleotides in DNA. By midsummer 1952, we knew that:
(1) The unusual nucleotide 5-methylcytidylic acid was to be found only in one isomeric form of one dinucleotide, which meant that it always preceded a guanylic in the DNA chain (this has proven to be general in the DNA of mammals and the presence of methylcydylic acid is now believed to be involved in the control of genetic expression);
(2) All of the other two-nucleotide combinations could be found in reproducible but varying proportions.
This latter was quite significant. One possible explanation of the Chargaff regularities (moles adenine = moles thymine and moles guanine = moles cytosine) had been that perhaps adenylic was always fol-
lowed by (or preceded by) thymidylic, and guanylic similarly by cytadylic. However, this clearly was not the case. The Chargaff regularities demanded another explanation.
I discussed this question with Fritz Schlenk, then professor of microbiology at Ames. The simplest explanation I could propose was that there must be two DNA chains, related in some way, so that wherever there was as an adenylic in one chain, there was thymidylic in the other, and likewise for guanylic and cytidylic plus methylcytidylic. This hypothesis had an obvious consequence, but unfortunately I was not then in a position to test it. If it were correct, then for every dinucleotide sequence, say CA, there should be an equimolar amount of the complementary sequence GT from the other chain (or TG if the two chains should have opposite orientation).
But our dinucleotides amounted to only one-sixth of the partial digest, and we could not then sequence the larger oligonucleotides. Nor could the dinucleotides present be considered a random sample as we were ignorant of possible preferences in the enzymatic digestion. Indeed, biochemical verification of this concept had to await the development of the "nearest neighbor" technique by Arthur Kornberg in his later studies of DNA synthesis.
At that time, I did not think in terms of helical structures and while I thought of the DNA as genes, I could not suggest any good reason for the presence of two such chains other than vague ideas about possibly coding for two proteins with some oddly defined relationship. My thoughts were oriented toward gene structure and expression, rather than toward gene reproduction and mutation, and so I missed the true significance of the complementary chains (so obvious when they are paired). This oversight was the result of an education that emphasized biochemistry and biophysics rather than inheritance and variation.
Shortly after I began my fellowship at Caltech in January 1953, I presented our dinucleotide data in a biochemistry seminar. I was somewhat surprised at the intense interest shown. I mentioned the two-chain notion, but, remarkably, the focus of attention was on the quantitative relationships among the dinucleotides, even though these accounted for but a fraction of the digest. I soon appreciated that the reason for this interest lay in prior discussions at Caltech about possible coding schemes to relate DNA sequence to protein sequence. Ours was the first quantitative data about any aspect of nucleotide sequence.
In February 1953, Linus Pauling announced a seminar at which he
would present the structure of DNA as deduced from X-ray diffraction data. As Pauling had earlier discovered the basic structures of proteins from X-ray diffraction data, his seminar was eagerly awaited. He put forward a triple helical structure for DNA with the phosphate groups on the inside and the rings of the nucleotides facing out. This structure was met with much skepticism. Many of the chemists present felt that the charged phosphate groups could not possibly be located in such close juxtaposition in the interior of the molecule.
Max Delbrück and I went to see Pauling to suggest possible modifications. Max was concerned that the chains of the helix should be paranemic (i.e., combable) rather than plectonemic (i.e., intertwined) so that they could be separated without the complication of unwinding—a very real problem for which the cell has had to devise special mechanisms. I wanted to propose that Pauling examine a two-strand helix to incorporate the Chargaff regularities and my notion of two complementary strands.
Pauling, however, could not be persuaded. He felt that his model was the only solution to the X-ray data and the known density of DNA. Unfortunately, as it later developed, he was using the old X-ray data and density data of Astbury, which had been obtained with a poor preparation of DNA that had been significantly denatured. Pauling had no access to the newer X-ray diffraction data of Franklin and Wilkins, which undoubtedly would have quickly set him on the right track.
The following month, Delbrück received a letter from Watson setting forth the Watson-Crick model. This immediately resolved many of the concerns raised about the Pauling model, which was abruptly discarded. The Watson-Crick model was then presented with immediate acceptance at the Cold Spring Harbor Conference in 1953. The convincing evidence for the biological, as opposed to the chemical, reality of the Watson-Crick model came from the Meselson-Stahl experiments at Caltech in 1957 demonstrating the separation and conservation of each of the DNA strands on replication.
The only substantial, if temporary, challenge to the Watson-Crick model came with my later discovery of the single-stranded DNA of the bacteriophage, f X. If Watson and Crick were right, how could this DNA reproduce or even code? The conundrum was subsequently resolved with the demonstration that, within the infected cell, the single-stranded viral DNA was quickly converted to a double-stranded replicative form, which replicated as such and which coded for proteins.
Later in the infective cycle, viral-coded proteins produced the single strands of DNA for progeny viral particles, using one strand of the double-stranded replicative form DNA as a template.
The Watson-Crick structure, by providing a firm biochemical basis for genetic phenomena, led biology into a new era, but it did not emerge unprecedented from a void. Mine was but one approach. Other lines of research in the laboratories of Kornberg, Doty, and Franklin and Wilkins were converging on the double helix. The double helix was out there waiting to be revealed. By 1950, after a century of biochemistry and fifty years of modern genetics, it was but a short step further in the unknown.
Science is, in fact, a collective enterprise. To outsiders, however, Watson and Crick by virtue of their great discovery and their strong personalities have made it seem a more personal adventure.
Jim Watson is the stuff of which People magazine is made. Brilliant, arrogant, verbally crude, with a skewed, off-center personality, fond of publicity, he sees the world in black and white with little gray in between. In his career, he has consistently demonstrated excellent scientific judgment and has been a superb director of the Cold Spring Harbor Laboratory. Crick is an interesting complement—highly articulate, sometimes glib, skilled in debate, sophisticated, and seemingly self-confident, even arrogant, yet harboring a persistent personal reserve.
Watson's book The Double Helix was published in 1968. Seemingly an exercise in candor, it portrays him as a total opportunist, devoid of any sense of scientific community, heedless in his passion to "beat Pauling" in the race to the "golden prize." Of course, Pauling never knew he was in a race. As a testament, it conveys an impression of science as just another cutthroat competition, akin to Wall Street or even the political arena. Shortly after the book appeared, I was awarded the California Scientist of the Year prize by the California Museum of Science and Industry for our research in collaboration with Arthur Kornberg at Stanford. At the inevitable news conference, reporters—with Watson's account fresh in their minds—could not comprehend the genuine, and really quite routine, collaboration between our two laboratories in the common effort to advance scientific knowledge.
11—
Iowa State—
At Full Speed
It was a rare privilege. Robert Oppenheimer and I were having lunch. He had come to Ames to deliver the first Frank Carlson Memorial Lecture, to honor his former student. We spoke of many things: the Institute for Advanced Study, which he now headed; the state of theoretical physics; the international situation; his long-standing interest in Hindu philosophy. He was curious as to the state of biophysics. I avoided mention of his recent "trial" at the AEC and his loss of security clearance—in my view a gross miscarriage of justice. He seemed to bear no rancor and his general mood was philosophical, detached, with an eye to the long view of events. I was captivated by his use of language—eloquent yet precise, almost poetically phrased.
I did not fully comprehend his subsequent lecture, which concerned calculation of the self-energy of the electron and discussed more recent refinements of work that Frank Carlson had initiated as Oppenheimer's student. But the luncheon discussion was a glimpse of true and wide-ranging brilliance.
After Caltech, my return to Ames was bittersweet—a homecoming, yet a letdown. Almost immediately, I went East to the Cold Spring Harbor Conference, at which the double helix structure for DNA was formally presented by Watson and Crick. After Caltech and Cold Spring Harbor, Ames seemed quiet—and, now, isolated.
As quickly as possible, I established my phage research. The bacterial viruses provided enticing access to a wealth of important biological problems. With them, genetic structure and function, gene replication
and mutation were all open to investigation with the several techniques we had been developing. These techniques were not perfect; they were limited and often tedious, but they would take us a long way. The full fruition of this was to come later with the research into the small bacteriophage f X174, but for the present I undertook to analyze the nucleic acids of the well-known T-series of phages.
There was a quiet moment of triumph when I observed my first phage plaques in Ames. My facilities were not comparable, but I had transplanted the phage technology from Pasadena to Ames.
To our astonishment, when we isolated the DNA from the T2 and T4 bacteriophages, we found that it could not be quantitatively digested to mononucleotides by the methods that had been successful with all other DNAs studied. It had been known that these particular viral DNAs had an unusual pyrimidine, 5-hydroxymethylcytosine, which replaced the usual cytosine. However, the resistance to the enzymatic digestion proved to be due to the presence of yet another, unsuspected, modification, a previously unknown pyrimidine. This was a glucose-substituted 5-hydroxymethylcytosine, which inhibited the enzymes. The extent of glucose substitution was 60 percent in T2 and 100 percent in T4; analysis of the progeny of phage crosses from mixed infections with T2 and T4 suggested interesting genetic interactions.
Many laboratories were working with T2 and T4 and these curious modifications of the viral DNAs spawned a variety of research projects, but I was soon diverted in other directions. As the research with f X progressed, it became more interesting and attracted an ever-increasing share of my attention.
After my experience at Caltech, I realized the importance of being informed of the most recent work in the field, which was now beginning to advance much more rapidly. Most of the research would of course ultimately appear in publications, but only after a delay of six months to a year. I had been a regular attendant at the summer Gordon Conferences; I now joined the American Society of Biological Chemists to be able to participate in its meetings, but these also were annual events. I maintained contact with colleagues I had met at Caltech. Also, by now some of our work had been published. This resulted in invitations to lecture at various universities and these visits broadened my network.
Through these activities, I became aware of the growing availability of support from the National Institutes of Health and the National Science Foundation. This possibility met a critical need. Having dem-
onstrated that the RNA of the tobacco mosaic virus could be isolated as an intact molecule, and accepting the new dogma that nucleic acids were invariably the genetic material, I was led to the concept that the RNA of the virus might by itself be sufficient to induce infection, without any involvement of the protein component. Indeed, we were able to demonstrate infectivity with purified RNA preparations. However, per molecule, the RNA was about 1/1000 as infective as the intact virus. By chemical means, we could not exclude the possibility of residual protein equivalent to a 1 percent intact virus contamination of our RNA preparation. We needed a means to purify the RNA more completely.
This could be done in an ultracentrifuge, which could separate molecules by size. But we had none; indeed, there were none in the state of Iowa, and since they cost twenty-five thousand dollars, the campus could not provide one for us. I therefore applied to the NSF for an equipment grant, and in due time received the funds and installed the first ultracentrifuge in Iowa.
Then, just as we set out to use this instrument to provide data to verify our concept that the RNA itself was infective, a paper from the Virus Laboratory at Berkeley announced their results, indicating the infectivity of TMV RNA. Competition in the field, and from better-equipped laboratories, was clearly becoming more intense. We were not entirely satisfied with the quality of the evidence in the published paper and undertook a series of experiments that definitively correlated the infectivity in the RNA preparation with the sedimentation of the RNA in the ultracentrifuge. Nice, even elegant, work but in effect a gloss.
There had been only the most rudimentary studies of X-ray diffraction patterns from RNA. Having in hand this large, homogenous, biologically active RNA led us to undertake such studies. Of course, we hoped to find another striking regular structure such as the double helix. Unfortunately, as we now know, most RNA does not assume such structures. While we obtained some valuable information about nucleotide and internucleoude dimensions in the RNA, no dramatic structure resulted.
Because of the progress of our work and the gradual change in its emphasis toward more functional aspects, I began to attract graduate students from the biochemistry program, which was formally located within the Department of Chemistry. To facilitate this interaction, I was given a joint appointment with the Department of Chemistry. Having this dual affiliation had some advantage but, as I soon found out,
also involved me in twice as many committee meetings and departmental disputes. But I enjoyed the personal contacts and could work within another domain to seek to increase the number of faculty doing work on the problems of what was coming to be called "molecular biology." I needed more colleagues, both for direct interaction and for their networks of professional acquaintance.
In the years 1953 to 1960, Max Perutz and John Kendrew develop the isomorphous replacement method of X-ray diffraction analysis and apply it to obtain the first detailed three-dimensional structures of the proteins myoglobin and hemoglobin. These structures incorporated the earlier models of Pauling and Corey but also provided specific details, down to atomic dimensions, essential to our understanding of the functions of the proteins.
As the genetic material, DNA must be accurately reproduced at each cell division. This reproduction is accomplished by a set of enzymes, DNA polymerases, which therefore play an essential role in heredity. In 1956 Arthur Komberg isolates the first DNA polymerase, making it possible to study DNA synthesis outside a cell.
The hereditary information of DNA is contained in the sequence of its nucleotide subunits. This information is used in the cell to specify the sequences of amino acids in proteins. Translation from nucleotide sequence to amino acid sequence is accomplished in a complex chain of reactions, in which a key role is played by "transfer RNA" molecules. These molecules, with the help of enzymes, recognize both a nucleotide sequence and its cognate specific amino acid. Mahlon Hoagland and Paul Zamencik first describe transfer RNA in 1957.
12—
Transition 3
At Ames in the early 1950s, I had the opportunity, indeed the luxury, to initiate and develop my research program at my own pace in relative isolation, drawing on my education and my imagination to explore an almost virgin field of great potential significance. In the expensive, intensely competitive, high pressure, teamwork world of molecular biology today, that would be impossible. Even by the mid-1950s, the field was attracting increasing attention and numbers, the pace of research was quickening, and isolation was no longer a boon but an increasing impediment.
In June of 1956, a major conference was held at Johns Hopkins University on the subject of "The Chemical Basis of Heredity." All of the leaders in the field were speakers and I was invited. At a break in an afternoon session, George Beadle steered me aside for a conversation. As was his custom, he came directly to the point. Would I be interested in moving from Ames to Caltech? I was pleased and surprised, but not totally. There had been subtle hints in recent correspondence with Delbrück, phrases to the effect that we might be seeing more of each other. My response to Beadle was yes, I would be interested, but my answer would of course depend on the specific conditions.
That fall I was invited to speak at Caltech at the dedication of their new Gordon Alles Laboratory. I spoke on "First Steps toward a Genetic Chemistry," reviewed the available knowledge of the chemistry of genes, and outlined the current problems and prospects for further advance. I also suggested that biology was entering a new era in which
our science was at last beginning to acquire the breadth and depth of information requisite to a molecular understanding of complex biological processes. I used as an example the recent elaboration by X-ray diffraction of the atomic structure of the protein myoglobin by Perutz and Kendrew. The first analysis of such structures, this remarkable achievement in one step increased our knowledge about this protein by several orders of magnitude. I saw this as the harbinger of the influx of information that would surely, and necessarily, become available with newer techniques and instruments.
The talk was published as a lead article in Science and generated hundreds of requests for reprints. It was clear that this field was flourishing and was about to burst into scientific prominence.
Somewhat to my surprise, no further mention of Beadle's suggestion was made on this occasion and I returned to Ames rather perplexed. But not for long. Within a few weeks a letter arrived with a formal offer from Caltech. I responded with some questions about laboratory space and equipment and meanwhile, as was expected, informed my department chairman of this offer. The central administration of the campus had changed in the prior year. The new dean and provost called me in to discuss my situation. It seemed clear to me early on that they would like me to remain at Ames and would raise my salary to match, nearly, that which Caltech offered, but that they did not really expect I would decline Caltech. And they were right.
Academically and scientifically, the decision was easy. On a personal level, however, it was difficult. We had sunk roots in Ames. We had many close friends, our children had schoolmates. We had an established and increasingly active niche in the community. It was hard for me to break these bonds, harder still for my wife and children who could see little compensating benefit. Most distinguished scientists have moved, often several times, in their career. Such moves are most often professionally beneficial, but there is always a price to be paid. Deep friendships wane to acquaintance. Life becomes a discontinuous journey.
My acceptance of Caltech's offer was accompanied by some trepidation. I had proven myself at Iowa State. Was I really in the same class as the eminent scientists I knew to be at Caltech? Well, it seemed they thought so.
For reasons still obscure to me, after I had made my decision, I fell, for the first and only time in my life, into a depression. It lasted some two or three weeks. I knew at the time, each day, that it was abnormal,
but I could not evade it. In this state, nothing seemed worthwhile. I went through the daily motions of existence, but life was totally flat and bleak. There was no joy, food had no taste, music did not please, the world seemed tinged with gray, all activities required effort beyond their worth. Worst was the sense, as day followed day, that this mood—unsought, undesired, unanticipated—might persist indefinitely.
I mention this because depression, like love and joy and anger and other emotional states, is only a word until it is experienced. Only with the experience can one understand and empathize.
As suddenly as it came, it lifted. The future—Caltech—was bright, truly bright, and I eagerly began plans for the transition.
13—
f X
The genes were the goal and viruses were to be the key. Viruses bridge the boundary between the living and the nonliving. As do all life forms, they reproduce and undergo inheritable variation (mutation), but, lacking any metabolism, they can only do so within the confines of living cells.
Viruses were discovered toward the end of the nineteenth century as mysterious agents of infection. Invisible under an ordinary microscope, their features could not be discerned until the invention of the electron microscope. Many varied forms are known that can infect animal or plant or bacterial cells. Max Delbrück first recognized that viruses—in particular, bacteriophage, which infect the simpler bacterial cells—afford the potential for detailed analysis of the processes of reproduction and mutation.
Genes, known since the time of Mendel to be the determinants of hereditary traits, were for decades biochemically utterly obscure. Early on, they were thought, by a process of elimination, to most likely be protein. No other structures seemed sufficiently complex. As the true dimensions of the nucleic acids became more apparent, several lines of indirect evidence moved them, in some minds, into contention for the genetic role.
Whether proteins or nucleic acids, the properties of genes seemed nearly inexplicable. How could a complex substance, of whatever chemical form, be reproduced with high precision through thousands of cell generations? Even more strange was the fact that, when a gene was
altered, mutated, by some unknown process, the mutant form was then reproduced just as faithfully as the original. And the mutant form could in turn be mutated further—or restored to its original state. Some, including Delbrück, proposed that a new principle of nature, a new physical law, might be needed to account for these phenomena. This speculation proved to be wrong—the ordinary principles of chemistry proved to be sufficient—but the proposal stimulated much discussion and fruitful research.
The choice of the bacteriophage-bacterium system proved to be most felicitous, providing a continual stream of new insights over a period of some forty years. In this period, the tools of molecular biology were developed and the study of bacterial virus infection provided a straight-forward and rewarding field for their application and refinement. The simpler viruses proved to be only a coated set of specialized genes. By simultaneous infection of a population of bacteria, one could initiate within these cells a completely novel sequential pattern of genetic activity that could be dissected into discrete steps.
My research on the bacterial virus f X174 over a period of twenty-three years was a significant component of these advances, sometimes leading the stream, sometimes being borne by it. It was the centerpiece of my scientific career and is illustrative of the spectacular advances in our knowledge during this time.
Our aim was bold. We were to use this virus to pry open the processes of heredity and infection. We sought to find its genes, to count them, to deduce their mode of action, to understand their reproduction, to learn the basis for their stability and mutability, and to identify the agencies of their control. To know the genes of even one virus this well would be of profound importance in itself, as all life is related and the principles we found should be general. Knowing the genes would lead to understanding the nature of viral infection, to learning how the synthetic machinery of a cell is subverted to the production of more virus. And this might, in time, provide a basis for therapy of viral disease.
Initially, we had only the rudimentary knowledge that f X was probably small and could grow in certain strains of E. coli . At the end of our research, we knew the complete sequence of its DNA and the details of its genetic structure and had an extensive if incomplete picture of its architecture and the processes of its replication. This was a classic period for virology, especially bacterial, and research on f X was one of the central features. But the path was not straight, the way was often uncertain, and there were many surprises en route.
During my six months in Max Delbrück's laboratory in 1953, I had decided that while bacterial viruses provided in principle the simplest system in which to study DNA structure and expression, significant advance (using the techniques of that day) would require the use of the simplest of the bacterial viruses available. The T-even bacteriophages, then under the most intensive study, seemed much too complex to me. Proceeding on the (partially erroneous) assumption that the reproduction of smaller viruses would be simpler to analyze, I scoured the literature in search of small bacteriophages. Two—f X174, discovered in Paris, and S13, discovered in England—seemed suitable. The evidence as to their size was only qualitative and insecure, for each line of evidence could have alternative explanations. But these viruses seemed a good place to start. Remarkably, cultures of each of these were available in laboratories in France and England, and an available strain of E. coli proved to be a suitable host cell.
After my return to Ames, I began work on these viruses in 1953. Work with any new virus requires that it be "domesticated"—that one learn the better media in which to cultivate the host and the virus, the conditions suitable for its storage, how to purify it, and so on. In early experiments, f X proved hardier than S13, which lost viability rapidly on storage. From then on, we concentrated on f X though, as we later learned, these two viruses are in fact closely related.
After learning how to produce cultures of high infectivity, we increased the scale of culture to provide quantities (in milligrams) sufficient for purification. The most useful techniques involved various forms of centrifugation. After some stages of purification, we were able to associate infectivity with a particular component that, because it moved relatively slowly under centrifugal force, supported our hope that the virus would be small. I then examined, in the electron microscope, virus from preparations that appeared nearly pure in the ultracentrifuge. Small, approximately spherical particles about twenty-five to thirty nanometers in diameter appeared, providing evidence that the total mass could not exceed eight to ten million daltons.
All viruses, of the small number that had been analyzed, were known to contain a nucleic acid, either DNA or RNA. We assumed f X would too, but which would it be? No RNA bacteriophage were then known, but animal and plant viruses containing RNA were common. After trying several methods, we were able to separate the viral protein from its nucleic acid and demonstrate that the latter was DNA. But an oddity. was immediately evident; its rate of movement under centrifugal force
was such that were it a conventional double-stranded DNA, its molecular weight would exceed that of the entire virus. How could this anomaly be resolved?
The research to this point had required three years. I reported it at the ASBC meeting in April 1957.
Research was interrupted for a time by my move to Caltech in the summer of 1957, but I resumed the phage studies as soon as possible.
I set up a light-scattering apparatus at Caltech that permitted me to ascertain first the absolute molecular weight of the f X virus particle (6.2 million daltons) and then that of its DNA, which proved to be 1.7 million daltons. This molecular weight, together with its centrifugal and other properties, suggested strongly that the DNA could not be the usual stiff, double-helical form. Could it be a single-stranded DNA, then unknown in nature? Or more likely, was it a denatured, crumpled form of a double-stranded DNA in which the stiffening bonds between the strands had been disrupted somehow by the extraction procedure?
Several lines of evidence suggested that the state of DNA inside the virus particle was no different than that we observed after extraction. But the convincing fact proved to be the quantitative determination of the nucleotide composition of the DNA by the methods we had developed in our earlier work. This DNA did not have the Chargaff equalities of A = T and C = G. The DNA of f X had to be a single-stranded, completely novel form.
These results were published to some astonishment in the spring of 1959. In June of that year, in a lecture at the Brookhaven Symposium, I compared this discovery to that of "finding a unicorn in the ruminant section of the zoo."
In the six years after its formulation, the double helix structure of DNA had become dogma. It so neatly accommodated the known facts concerning DNA structure and replication that the proposed presence of a single-stranded DNA—in of all things a virus, a form specialized for reproduction—aroused amazement and doubt and stirred the imagination. Soon, many of the best graduate students at Caltech wanted to work in my laboratory, and I received numerous applications from postdoctoral fellows from around the world. Success breeds success, and the talent thus attracted surely facilitated further progress.
How could the single-stranded DNA of the f X virus reproduce? Was there another mechanism in addition to the mode of complementary replication implied by the Watson-Crick model of double-stranded DNA and demonstrated subsequently by the famous Meselson-Stahl
experiment? To study this question, we undertook to follow the fate of the viral DNA after it was introduced into the bacterial cell. This was a novel, even daring, proposition that called on several of the newly developed techniques of molecular biology. Many of these techniques—the use of nonradioactive and radioactive isotopes, light scattering, the various forms of ultracentrifugation—were based on specific applications of physical principles. My background in biophysics served me well. The small size of the f X DNA, which permitted us to manipulate it with minimal degradation, was also critical.
We developed three distinct means to identify viral DNA within the infected cell and to determine its integrity. The most rigorous of these required measurement of the infectivity of naked viral DNA. According to the most common but not yet universally accepted theory, the protein coat of the virus merely served to protect the DNA and assure its entry into the infected cell. Once inside, the DNA, the genetic material alone, was thought probably sufficient to carry on the infection and give rise to progeny virus particles. If this were so, the free DNA would be infective if we introduced it into a bacterial cell.
We were able to accomplish this by preparing, under appropriate conditions, bacterial protoplasts—cells that lacked a layer of their cell wall and therefore were somewhat permeable to the free DNA alone. George Guthrie, then a graduate student, developed this technique and was able to achieve an infective efficiency of one infected protoplast per one hundred to one thousand viral DNA molecules. This figure was low compared to that of intact virus, but it was sufficient for our purpose. Theory aside, this was the first complete demonstration that a viral DNA was sufficient for infection. Combining all of the techniques, we could infect a cell and subsequently identify, track, and assay the infective potential of the intracellular DNA molecules of the parental virus. We could isolate these at various times after infection.
These combined experiments demonstrated conclusively that the parental viral f X DNA remained intact and infective on infection of the host cell. But within one to two minutes it was converted to a different form of DNA, which we called the "replicative form." Physical and chemical studies of the replicative-form DNA demonstrated that in fact it was the double-stranded version of the single-stranded viral DNA, paired with its newly made complementary strand. This replicative form was also infective to protoplasts. Further studies revealed that, after its initial formation, the replicative form reproduced as such, in the usual semiconservative manner of double-stranded DNA to produce some ten
to twenty copies. This set of replicative-form DNA molecules then served as the template from which the distinctive single-stranded DNA of the progeny virus particles was made during the latter half of the infection. So replication of this single-stranded DNA in fact proceeded by the usual, complementary strand mechanism, albeit with some atypical aspects.
However, another major surprise was now in store for us. We had available from f X, for the first time, a homogeneous DNA of defined size (about fifty-four hundred nucleotides long) and nucleotide composition, with a specific biological activity (infection). It seemed a reasonable question to inquire whether there was anything special about the ends of this DNA. Was there a specific nucleotide or a specific sequence at either end of the chain? (Nucleic acid chains have a polarity and the two ends are distinguishable.) We had available two enzymes that we knew would degrade a polydeoxynucleotide chain from respectively one or the other end taking off one nucleotide at a time. Walter Fiers, a postdoctoral fellow, undertook to study the effects of these enzymes on the purified viral DNA and to characterize the products of the enzymatic action.
His results, repeated many times, were surprising. When he used highly purified enzymes, only a small fraction of the viral DNA appeared as mononucleotides, and these included all four possible in similar proportions. Further, there was no perceptible decline in the infectivity of the DNA after the enzyme digestion. Clearly, the bulk of the viral DNA, all of the infective DNA, was resistant to the action of these enzymes. Why? Were both ends of the DNA "blocked" by some special chemical group?
One day while walking back to the laboratory after lunch at the Athenaeum and pondering this question, I began to consider seriously a possibility we had occasionally tossed off in jest that the DNA might really be a ring—not a linear molecule, but a ring. A ring would of course have no ends to degrade, but if it were a ring it would behave slightly differently—it would move slightly faster under centrifugal force—than would the corresponding linear DNA of similar weight. Now in fact, in all of our DNA preparations, we had actually observed two components in the ultracentrifuge with closely similar rates of movement, present in varying amounts in different preparations. Preparations with the greater proportion of the faster-moving component had appeared to have higher specific infectivity. Our more recent preparations, made with better technique, had only small amounts of the
slower component We had had no explanation for this observation.
If the viral DNA were a ring, which would be the faster component, then the slower component could be rings that had accidentally been cleaved, linearized, at some random point and were therefore noninfective. Further, the ends of such randomly cleaved rings could account for the small yield of terminal nucleotides of all four kinds that we had observed on enzyme treatment.
An experiment to test this hypothesis became clear to me. I would start with a preparation of (almost) pure rings, then cleave them deliberately and slowly with an enzyme that would break the chains randomly. The proportion of rings cleaved could be followed by the loss of infectivity. As the rings were cleaved, DNA molecules would be transferred, first from the faster component to the slower component and then, as a second cleavage was made, out of the slower component into smaller fragments of random size.
The following afternoon I worked out the equations relating residual infectivity to the proportions of DNA in the first component, second component, and smaller fragments. Walter Fiers performed the experiment; it precisely confirmed the theoretical expectation. f X DNA had to be a ring.
I still consider this experiment, combining measurements of biological infectivity, ultracentrifugal analysis, and enzymatic activity, as probably the most elegant with which I have been involved.
The result, which I thought unarguable, met initially with considerable skepticism, particularly from nucleic acid biochemists. They were wedded to the concept of a polymer that, starting at one end, was extended unit by unit to the other. A ring molecule provided no purchase for such extension. If the single-stranded viral DNA was a ring, was the double-stranded replicative form also a ring? Alice Burton, then a postdoctoral fellow, carried out the analogous experiment, degrading the replicative form with an enzyme known to cleave both strands at the same site. She thus demonstrated its conversion to a linear, slower moving form in equal proportion with its loss of infectivity.
Then came an opportunity to satisfy the skeptics—seeing is believing. Dr. Albrecht Kleinfelter, then at UC Berkeley, had developed a method to visualize DNA in the electron microscope. It could only, however, be applied to double-stranded DNA. I took some replicative-form DNA to his laboratory, and we prepared the electron microscope grids and took pictures. When we examined the small pictures with a hand lens, the very first DNA we saw was a ring. Indeed, the field was filled with
double-stranded DNA rings of the circumference to be expected for the known weight of DNA. The skeptics were convinced.
Later, when it became possible to visualize single-stranded DNA in the electron microscope, the rings of the viral DNA were quite obvious.
Why had this result been so hard to deduce? In retrospect, we had been mesmerized by the predominantly linear character of DNA. To have a ring, one had to have an intact DNA. Prior to f X, everyone had studied fragments of DNA. Since then, many other ring DNAs have been found in viruses, plasmids, mitochondna, chloroplasts, and so on. In fact, it has become clear that for those DNAs that are not rings (as in eucaryotic chromosomes) special means must be provided to replicate their termini.
Now we had a complete DNA molecule, the complete genome of this virus. It was all there in our test tube. But how did it multiply? How did this relatively small molecule subvert the machinery of the infected cell to achieve its own reproduction?
Biochemically, the next logical step in our research would have been to determine the nucleotide sequence of the viral DNA, which could plausibly be expected to lead us to the genes through the genetic code then being deciphered. However, the technology to do this was not then available. We were able to separate out all the pyrimidine nucleotide tracts from the intervening purine nucleotide tracts and then to fractionate these according to size (thus learning that the longest consecutive run of pyrimidine nucleotides was eleven), but we could neither further fractionate nor sequence these tracts. So the information gain was limited.
We turned, therefore, to genetics. Using the recently developed methodologies for conditional lethal mutations (i.e., mutants able to grow under one set of conditions or in certain cells but unable to grow under other conditions or in other hosts), we were able to obtain a large number of mutants of f X. These could be classified into "complementation groups," or genes, by determining whether a successful infection would ensue in a cell infected with two different mutants. If so, then each mutant must supply the function defective in the other so that they were in different complementation groups. If such a joint infection were not successful, it was plausible that the mutants were from the same complementation group, that is, defective in the same gene. Nine complementation groups were discovered and named A–H and J. The mutations could be mapped—arranged in a linear order along a (necessarily circular) path—by the usual means of recombinant formation in genetic
crosses. Only the mutants in genes D and E could not be consistently ordered for reasons that much later became apparent.
Studies of the abortive infective process in cells infected with these mutants provided clues as to the functions of the several genes. Gene E, for instance, was required for disruption of the cell membrane at the end of the infection, thereby releasing the progeny virus. In gene E mutants, the membrane failed to disrupt and viral synthesis continued extensively. As we could then artificially disrupt these cells, these mutants were useful to provide high yields of virus. Some genes proved necessary for early stages of DNA synthesis. Others were crucial for intermediate stages of viral particle assembly, while others coded for the four proteins of the viral coat.
Parallel with these studies, a long-term collaboration with Arthur Kornberg of Stanford proceeded intermittently. Professor Kornberg, a distinguished enzymologist, had devoted years to the biochemistry of DNA synthesis and had isolated and purified an enzyme (DNA polymerase) from the E. coli bacterium that could synthesize DNA using as a template whatever DNA it was given to copy. While chemical analysis indicated that the enzyme was making faithful copies, the ultimate test would be to demonstrate that the copy DNA had a true biological activity. With its defined size and a functional assay (infectivity), f X DNA was an obvious choice to test the faithfulness of Kornberg's enzyme. Could it make infective DNA copies in vitro?
Using our DNA, Kornberg's enzyme, and our infectivity assay, we tried in 1960. While considerable amounts of DNA were made in excess of that initially added, infectivity fell rapidly. Attributing this result to impurities (degradative enzymes) in the DNA polymerase preparation, we tried the experiment again in 1963 with a more highly purified enzyme. The same result.
With the discovery that f X DNA was a ring, it became evident that effective synthesis would require an agent to close the newly made DNA strands into a continuous circle. A recently discovered enzyme, DNA ligase, had the ability to do this.
In 1967, with the help of Mehran Goulian, a postdoctoral fellow in Kornberg's laboratory, we set out again to make infective DNA using DNA polymerase together with DNA ligase and f X DNA. To insure that the synthesized (and, we hoped, infective) DNA was distinct from the input viral DNA, we incorporated a density label so that the product could be physically separated from the template. The experiment was eminently successful. Together, we had made for the first time an in-
fective agent in the test tube capable of thereafter reproducing itself indefinitely in host cells.
In truth, we had only copied faithfully an existent design. But our copies were, in fact, potentially immortal.
We reported this work in the December 1967 issue of the Proceedings of the National Academy of Sciences . By some circumstance, this report landed on the desk of an aide to President Johnson on the day the president was to speak at the National Institutes of Health. It was incorporated into the president's speech as "the nearest approach to the synthesis of life in a test tube," an instance of the remarkable research supported by NIH. As a consequence, this result was reported in newspapers and news magazines everywhere. A result of such coverage was the receipt of many letters, some from long lost friends or relations, some quite touching. The newspaper articles, referring to the small size of f X, sometimes called it a "dwarf virus," and I received several letters from people suffering from dwarfism who asked if my research could alleviate their affliction Sadly, not.
In the early 1970s, our ability to analyze DNA was greatly augmented by two developments. The restriction enzymes were discovered, which cleaved DNA only at specific nucleotide sequences. Using these, a DNA such as that of f X could be broken reproducibly into a small number of specific, isolatable regions. By the use of partial digests, Amy Lee in my laboratory was able to order these regions into a continuous map, the first complete restriction fragment map of a DNA. By hybridizing one or more of these fragments with the viral DNA, Lee Compton was able to visualize specific regions of phage DNA in the electron microscope.
Even more significant, Fred Sanger at Cambridge University was developing means to sequence the DNA of restriction fragments nucleotide by nucleotide. As a defined DNA of specific size with an ordered restriction fragment map, f X was the natural choice for Sanger's first application of this technique. After obtaining his Ph.D. in my group, Clyde Hutchison went to Sanger's laboratory to help with this project. In 1977, Sanger announced the complete nucleotide sequence of f X, 5,386 nucleotides. This was the first complete sequence of any DNA, a major accomplishment. As such, it marked the end of an era of f X research and the beginning of a vast new period of DNA research.
Sanger's sequence indicated clearly the location of the genes we had laboriously sited on the genetic map. It also contained one major surprise. We had been unable to map genes D and E in a consistent man-
ner; the reason now became clear. The two genes used in large part the same region of DNA, reading it in different, overlapping trinucleotide frames!
This possibility had been discussed before but was usually dismissed with the argument that such an overlapping pattern would result in such great constraints of the choice of amino acids that it seemed very unlikely that two functional proteins could result. Yet here it was!
In small viruses such as f X, the physical constraint on the size of the DNA evidently drives the virus in an evolutionary sense to make the very maximum use of its fifty-four hundred nucleotides. Other small overlaps, at the ending of one gene and the start of the next, attest to the same pressure, with the use of one region of DNA to code for portions of two proteins.
At this same time, f X was also serving an important role in the attempt in Arthur Kornberg's laboratory to reconstruct from E. coli extracts an entire system able to initiate, extend, and complete DNA synthesis. The use of f X DNA as a small, defined template enabled this laboratory to extract and successfully refine the many factors needed, in addition to the DNA polymerase and ligase, to reproduce DNA in a truly biological fashion. Out of this work has come the recognition that while the smaller viruses, with less genetic material, contribute fewer components to the infective process, the host cell must therefore contribute more. In brief, the smaller viruses are the more parasitic; their infective process, resulting in viral reproduction, may not be appreciably simpler than for larger viruses.
While certain aspects of f X replication, in particular the manner of particle assembly, remained obscure, by 1977 f X had fulfilled its primary role in the advance of molecular biology. My original conception in undertaking f X studies—the need for a simple, defined, biologically assayable DNA as an experimental subject—had been validated. The research with f X had played a significant role in a classic period of advance in our understanding of genes. The technology was now available to attack more complex problems.
This tale—the unraveling and elucidation of the structure and process of replication of one virus strain—can serve as a prototype for much of biological research. Starting with but a few empirical observations, each finding led to deeper questions of detail or process. To answer these questions required the development, application, and refinement of new techniques and new approaches. Some came from our laboratory; others were borrowed from Kornberg's laboratory, or Sanger's,
or were culled from the literature on restriction enzymes, conditional lethal mutations, or ultracentrifugation. Out of this study of one virus came often surprising discoveries of great generality—that there are viruses containing single-stranded DNA; that many DNAs are rings; that single-stranded DNAs reproduce via the conventional complementary mechanisms; that, in small viruses, the same DNA region can be used to determine more than one protein—as well as the important demonstrations that an infective DNA can be synthesized in vitro and that a complete genome can be sequenced.
These are the rewards of a sustained, collective effort: meaningful contributions to human knowledge. They were not easy won—one does not write of the failed experiments, the misconceived, the accidents, the equipment failures, the blunders. One cherishes the nuggets of knowledge, and the students who mastering new skills on these problems have gone on to make advances in many other fields.
14—
The Caltech Years—
The Fulfillment
My first decade at Caltech was exhilarating. Not only was the f X research progressing steadily and successfully into previously inaccessible processes, but more broadly it was a breakthrough era for molecular biology. The many ideas, hypotheses, and concepts immanent in the double helix model for DNA were about to receive experimental test and verification or refutation. Within the next half dozen years the "semiconservative" model for DNA replication was demonstrated, the genetic code was deciphered, and "messenger RNA" was discovered as the intermediate in the conversion of genetic information into protein structure. Caltech played a significant role in each of these advances.
The invention and theoretical analysis of the technique of density gradient centrifugation by Jerome Vinograd at Caltech provided the methodology for the brilliant experiments of Meselson and Stahl, as well as some of our own crucial f X experiments. Using the stable heavy isotope of nitrogen (N15 ) as a density label, Meselson and Stahl were able to demonstrate that, on replication of DNA, the two strands of the double helix dissociate; each strand, remaining intact, becomes one of the two strands of each daughter double helix. This process had been postulated but, as we were completely ignorant of the complex enzymatic processes involved, had remained in doubt until experimentally verified.
The correspondence between DNA as gene and protein as "gene product" was clear, but the nature of the biochemical connection was unknown. Progress in the achievement of protein synthesis with extracts
from disrupted cells had indicated that proteins were actually synthesized on subcellular particles called ribosomes. But the ribosomes of a cell were chemically uniform. How then were individual ribosomes programmed to produce different proteins? Experiments in several laboratories, including particularly research conducted by Brenner, Jacob, and Meselson at Caltech, demonstrated that a minor, ephemeral variety of ribonucleic acid, called "messenger RNA," was made by copying into RNA portions of the cellular DNA. Such messenger RNA molecules then served for a short time as programs to be read by the ribosomes, to produce the specific proteins.
Clearly, the genetic information contained in the sequences of nucleotides in DNA, as copied into the messenger RNA, somehow specified the sequences of amino acids in the various proteins. But the code connecting these two sequences was unknown. Delbrück was very interested in this and there was much speculation and many seminars about the nature of the genetic code: overlapping codes, self-correcting codes, commaless codes, two-letter, three-letter, four-letter, six-letter codes were discussed with proponents of one or another variety proclaiming its apparent or unique merit. It was a game many could play and it seemed that if one were really clever, one could deduce the one clearly optimal code that nature had chosen to use.
But the experimental problem of deciphering the actual code seemed difficult to resolve until the highly fortuitous discovery by Marshall Nirenberg that, under nonphysiological conditions, protein-synthesizing systems extracted from cells could use artificial polynucleotides as messenger RNAs. Such synthetic polynucleotides could be made of very simple sequences, resulting in the synthesis of correspondingly simple chains of amino acids. The obvious correlation then of amino acid sequence with nucleotide sequence permitted the complete decipherment of the genetic code within a few years.
At this same time, the discovery of the "amber" viral mutants by Richard Epstein at Caltech—mutants that could only grow in cells that used a slightly altered genetic code—opened a wide new path to the identification of specific genes and the analysis of their function.
It was an heroic time in which individual scientists working with small groups could, with great effort and ingenuity, make momentous discoveries. These discoveries, over a space of little more than a dozen years, transformed biology by exposing the molecular machinery of genetic reference, thereby prying open and making accessible the basic processes of life.
It is hard now to realize how difficult those first steps were, how months of step-by-step experimentation and correction were required to obtain a result that could now be achieved in a few hours. But it was accomplished, and those involved will always remember the thrill as each new discovery, was announced, each new perspective revealed.
After these discoveries of the 1950 to 1965 period had been consolidated, some, notably Gunther Stent, proclaimed the end of the "golden age" of molecular biology: all of the great discoveries had been made and, most disappointingly, no new fundamental principles had been discovered; biology turned out to be a specialized form of chemistry. But Stent's vision was woefully short. He did not foresee the second and even greater flowering of molecular biology.
The invention of recombinant DNA in the early 1970s by Herbert Boyer and Seymour Cohen enabled the extension of molecular biology techniques far beyond the small discrete nucleic acids of viruses to the much more complex nucleic acids of higher organisms. And the development of methods for the determination of nucleotide sequence in DNA by Walter Gilbert and Fred Sanger improved the effective discrimination of these techniques by orders of magnitude, permitting the detailed analysis of mutation and genetic control mechanisms. The previously inaccessible fields of developmental biology and physiological control then became open to analysis at the molecular level.
In this same period, Caltech was the scene of other remarkable scientific accomplishments. Martin Schmidt and Jesse Greenstein discovered quasars, the intensely powerful and distant sources of radiation that are believed to be at the centers of galaxies. Murray Gell-Mann developed his quark model of the structure of subatomic particles, which brought coherence to an increasingly diverse and puzzling set of data. The Jet Propulsion Laboratory, after some difficulties, successfully sent the first probes to land on the moon.
Richard Feynman in physics was the one true genius I have known. He had an unsurpassed depth of understanding of the fundamental principles of physics in the broadest sense. I watched in astonishment as, on more than occasion, he swiftly applied this deep insight to completely novel situations and assorted and arrayed the important variables in some comprehensible manner.
What special qualities made Caltech the locus of many of these advances? Caltech is unique. A small, private school, focused on basic science and advanced engineering, it attracts the brightest freshmen (as
measured by SAT scores) of any college or university in the country. Because the freshmen class is limited to 220 students, now about 25 percent female, the resultant undergraduate student/faculty ratio of three to one permits individual student recognition. At the same time, the faculty is able to devote most of its time to research, working with some one thousand graduate students and four hundred postdoctoral fellows. The concentration of interests, the uniformly high level of talent, and the opportunity for close interaction with faculty make it an excellent school for students who know their educational goals. For others its opportunities can be too limited.
The renown of the institute has enabled it to develop extensive funding and it has the highest ratio of endowment per faculty member of any private university. Its ability to provide substantial research resources in support of individual faculty members has in turn enhanced the ability of those members to achieve and maintain eminence in their fields.
Absent the elemental necessity to hire enough faculty to provide the needed classes for large numbers of students, Caltech can be highly selective in its choice of permanent faculty. Similarly, as a private institution, Caltech can be highly selective about the fields of education and research it chooses to undertake. This freedom to focus energy and resources on a few specific areas within, for instance, biology or physics has permitted the institute to deliberately select the most promising frontiers in any period and to develop a "critical mass" of interactive faculty to explore and exploit those fields.
Careful screening among the most promising applicants for junior faculty positions is followed later by application of rigorous criteria for the award of tenure. As a general principle, tenure is awarded only to junior faculty members who have demonstrated accomplishments sufficient to place them among the "top five" in their field and age group in the country. In consequence, 21 percent of its faculty are members of the National Academy of Sciences and 8.5 percent are members of the National Academy of Engineering.
The small size of the Caltech faculty facilitates scientific contacts among the several disciplines. Such interaction is fostered by the presence of a rather elegant faculty club, the Athenaeum.
The Athenaeum often provided an intellectual feast, a gourmet meal for a science junkie. One might talk with a geologist about the age of the earth, the composition of moon rocks, or the temperatures of ancient seas. Or with an astronomer about the precise periods of pulsars
or the mysteries of quasars. Or with a physicist about the zoo of fundamental particles, the oddities of quarks, or the postulated gravity waves. Or with an engineer about supersonic planes and rockets, space exploration, lasers, or the potential of computers. Or with economists or historians to hear their perspectives on the events of the time. To be sure, there was always politics or the fortunes of the Dodgers to fill in the gaps.
As compared to MIT, Caltech is much smaller, with about one fourth the student body. Caltech is much less diverse, with a narrower spectrum of engineering disciplines and no schools of architecture or management. Basic science plays a larger and engineering a lesser role. The breadth and scale of MIT permit it to consider problems of large societal concern and impact such as transportation, urban planning, and industrial competitiveness.
Caltech is quite comfortable with itself in its present format. It has a self-image of singular superiority, which is not quite as readily justified as formerly. For, while still unique, Caltech is not as peculiarly distinctive as it was in an earlier era. Other, much larger institutions have developed concentrations of faculty in one or more specific areas able to rival those at Caltech. But it remains an extraordinary constellation of excellence in its chosen sectors. While the limitations imposed by its narrow focus on curricular offerings and research opportunities are recognized by some, the institute has not been sufficiently motivated to undertake the major effort required to transcend them.
When we arrived in the 1950s, Pasadena still had a small-town atmosphere. Unfortunately, at times it also had intense smog generated in downtown Los Angeles and blown up against the mountains. Nevertheless, there were pleasant residential neighborhoods within walking distance of the Caltech campus in which many faculty lived. We found a residence nearby that greatly facilitated return in the evening to initiate or complete laboratory experiments. This ease of access also facilitated attendance at evening lectures and engendered a sense of campus community.
The biology faculty at Caltech was, and continues to be, extraordinary. When I arrived, George Beadle was the chairman and unquestioned leader, a "father figure" to most of the department. When he subsequently became dean of the faculty and proposed that the department find another chairman because of the demands on his time,
we would have none of it. Only when he left to become president of the University. of Chicago did we replace him.
Henry Sturtevant was the "grand old man" of the genetic community. A student with Morgan in the early days of drosophila research at Columbia, Sturtevant had known personally almost all of the great figures in the history of genetics. He had an encyclopedic knowledge of genetics and entomology as well. As I saw him age and reach retirement, I realized how much is lost when such an irreplaceable mind is gone. Ed Lewis, his protégé, would later carry on the drosophila tradition with great distinction. Sterling Emerson, Ray Owen, Norman Horowitz, and Herschel Mitchell completed an extraordinary grouping of geneticists.
Frits Went with his Phytotron, in which he could grow plants under precisely controlled conditions, and James Bonner and Arie Haagen-Smit, who were more biochemically oriented, constituted an impressive program in basic plant biology. Haagen-Smit had already become interested in the chemistry of the pervasive smog problem and had deduced the primary chemical reactions involved. Meanwhile, Henry Borsook, a long-time biochemist, was pursuing protein biosynthesis, a problem that would only be solved subsequently by much newer approaches. "Case" Wiersma and Anthonie Van Harreveld were classical neurophysiologists engrossed in electrophysiology, while Roger Sperry undertook his brilliant and idiosyncratic studies of the factors determining neuronal connections.
All told, a remarkable group.
Caltech provided me the opportunity for research at a higher level of intensity and on a different scale than had Ames. Very able graduate students wanted to work in my laboratory; postdoctoral fellows from Europe, Asia, and the U.S., eager to have experience at Caltech, soon applied to study with me. Over the years, I had scholars from Great Britain, the Netherlands, Belgium, France, Sweden, Germany, Czechoslovakia, Hungary, the Soviet Union, Japan, and Chile work in my laboratory. It is a great pleasure now to be able to travel about the world and visit former students and see how well they are doing in their science in their native countries. Science is truly an international activity. I can walk into a lecture room in Kyoto, Santiago, or Stockholm and see the periodic table on the wall and feel at home.
And, as well, I regularly had one or two bright undergraduates, eager to begin research, working part-time with me.
Teaching responsibilities at Caltech were much different than at Iowa State. Formal classroom obligations were much reduced and there were no large "service" courses akin to engineering physics. However, there was much greater informal educational activity, of a more personal and focused nature, with graduate students and postdoctoral fellows in my laboratory and in small seminar-type courses in which a current topic of interest would be discussed in depth.
"Introduction to Biology," which was taken for one academic quarter by about half of the freshman class, was the largest biology course; I taught this for six years and enjoyed presenting the essence of the new biology to such bright students. In later years, I introduced a course in "Biology and Society" that considered the social and ethical issues arising from the advances in biology and medicine.
In addition to attendance at seminars and lectures, I sought to broaden my knowledge of biology by sitting in on various classes. Max Delbrück presented a one-quarter lecture course each year on a topic of his choice He took this quite seriously and used the course as an opportunity to learn about a subject of interest. One year he talked about biological membrane structure and function, another about basic solid-state physics and order-disorder phenomena, a third about transducers in sensory processes with particular discussion of the optic retina.
Caltech undergraduates are remarkable. Uniformly bright, with high analytical skills, they have a focused intensity that certainly surpasses the students of my MIT days. Perhaps because they are more oriented toward science than engineering, the student culture is less attuned to the larger world and thus more susceptible to the fascination of research and scientific discovery.
As one result of the exclusively technical emphasis at Caltech, life for students and faculty can be remarkably insulated from even major external political or social concerns. In the 1960s we observed the upheavals, riots, and drug scenes on such campuses as Berkeley and Columbia from a distance with dismay and some disdain. Caltech students were too serious and committed for such folly. But our own children were not immune to the peer movements of the time and through them we achieved at least some understanding of the youthful idealism and depth of feelings involved. And Vietnam reached even Caltech, however peacefully, with large discussions and teach-ins.
From the beginning, this military action had made no sense to me. Vietnam seemed to be primarily a civil war with which the U.S had no great concern. And as the war dragged on, with growing casualties and
an evident unwillingness in Washington to do what would be necessary. to win it, our involvement seemed ever more a cruel and grotesque mistake—one from which the nation would long suffer.
A senior faculty position at Caltech conveys a prestige that launches one into larger spheres of activity. Soon after I joined the institute, John Kendrew came through to see me about starting a new journal. Publication in the field of molecular biology was still hindered by the too-specific interests of existing journals. Out of this came the Journal of Molecular Biology with Kendrew as editor-in-chief and myself as one of the associate editors. It is today still a leading journal in the field of macromolecular structure and function. I served as an associate editor for ten years. After this, I served for five years as a member of the editorial board of the Annual Reviews of Biochemistry, and, subsequent to that, for eight years as chairman of the board of editors of the Proceedings of the National Academy of Sciences . Such service is important to the maintenance of the scientific standards of publication in the field; it also enables, indeed requires, one to keep up with the advances across a much wider sector of biology than one's own research interest. However, it requires a considerable investment of time and intellectual energy that is not usually professionally rewarded.
I was asked to chair—which meant to draw up the program and select the participants—the 1960 Gordon Conference on Proteins and Nucleic Acids. This was the last year when both fields could be considered together. Organizing such a conference is a considerable logistic task and the supporting services of an institution such as Caltech are essential. It is a pity that video records could not have been made of these Gordon Research Conferences in the 1950s and 1960s. It was here that great advances and important smaller steps were often first presented, discussed, and critiqued in the informal, open, and friendly fashion of science.
Here Fred Sanger presented the first amino acid sequence of a protein, insulin. The sequence of the adrenocorticotropic hormone was not far behind.
In the early days, DNA or RNA preparations from cells were, of necessity, treated as homogeneous substances. While, for many purposes, this proved to be valid for DNA, many years and many conferences were required to sort RNA into its various classes of messenger, transfer, and ribosomal RNA with different structures, functions, cellular locations, and turnover rates.
At the Gordon Conference, Julius Marmur and Paul Doty described
their remarkable discovery of DNA renaturation, the basis of much of today's molecular genetics. The enzymology of DNA and RNA was progressively elaborated. Here, too, Francis Crick presented his "adaptor" hypothesis to link nucleic acid sequence to amino acid sequence and his "commaless" code. Mahlon Hoagland and Paul Zamencik described their discover, of transfer RNA. Bacterial transforming factors and viral structures were analyzed and the relationship of DNA structures to genetic factors was progressively refined. Gradually, year by year, the modes of synthesis of DNA and RNA and protein were clarified.
I will never forget Seymour Benzer's succinct interruption of a prolonged presentation by Gunther Stent. Gunther, in a late evening session, was describing in endless detail a complex bacteriophage experiment that had yielded negative results. Finally Seymour spoke up, succinctly and loudly: "Big deal!" The meeting abruptly adjourned to the bar.
Each year the field progressed and expanded with new knowledge and new researchers. After 1960, the meetings had to become more numerous and more specialized.
In 1960 I received my first invitation to an international scientific conference at Chamonix in France and so had my first experience abroad. Europe was a daily surprise. We landed in Copenhagen and I was stunned to be in the midst of crowds of people all speaking a tongue that meant nothing to me. Of course —but it felt like a verbal assault. In Europe, at least I could read the signs in the streets and at terminals. A few years later in Japan, I realized what it must be like to be illiterate, to be totally unable to find one's way—or worse, even to ask.
We visited the major cities: Copenhagen, Brussels, Paris, Geneva, London. The wealth of architecture and art accumulated over the centuries was astonishing. London still bore the scars of the wartime bombing. Each distract sector of Paris entranced us. I was struck by the omnipresent weight and legacy, of the past, so different from the ever-changing life at home.
The remarkable progress in molecular biology received wide attention. Many universities had not kept up and now lacked faculty in this suddenly important area of biology. I began to receive unsolicited invitations from other universities, many quite distinguished, to join them, often accompanied by commitments to develop a major program in molecular biology that I would head.
At first I was flattered and considered a few of these sufficiently se-
riously to visit and learn of their plans and evaluate the opportunity. But I soon realized that I simply did not want to leave Caltech to be a professor elsewhere. I had all of the resources and opportunity I needed, I had first-rate colleagues, and I liked the living arrangements. Thereafter, although I continued to receive such invitations, I simply declined with thanks, not wanting either to waste their time or to stir up uncertainty in my own life. In a few instances, however, I fear my refusal even to look at their offer was misinterpreted as arrogance.
According to Watson-Crick double helix model for DNA, the two strands of the double helix should be separated upon replication of the DNA. In 1958, in an important test of the model, Matthew Meselson and Frank Stahl experimentally confirm this separation.
Following the discovery of the double helix structure, it was expected that all DNA would be double-stranded. It is thus a considerable surprise when, in 1959, the DNA of the virus f X is shown to be single-stranded.
In 1960, Paul Doty and Julius Marmur discover DNA renaturation, wherein if the two strands of a DNA double helix are separated in solution, they will subsequently pair up in precise register and reform the double helix. This process forms the basis for much of the technology of molecular genetics.
Also in 1960, Marshall Nirenberg produces, in vitro, a polypeptide translated by a cellular extract from a synthetic polyribonucleotide Further exploitation of this discovery by Nirenberg, Severo Ochoa, and Gobind Khorana leads to the complete elucidation of the genetic code linking nucleotide sequence to amino acid sequence.
DNA does not serve directly as the source of information for protein structures, but is first copied into another form of nucleic acid, called "messenger RNA." Messenger RNA then participates directly in protein synthesis. In 1961, Sydney Brenner, François Jacob, and Matthew Meselson first describe messenger RNA.
DNA was thought to exist exclusively as long linear strands. In 1962, the DNA of the virus f X is demonstrated instead to be a ring molecule, a structure since shown in many viral and other DNAs.
In 1967, Mehran Goulian, Arthur Kornberg, and Robert Sinsheimer reproduce an infective DNA (f X) in vitro This accomplishment confirms the validity of many of the biochemical procedures then in use and opens the door to the test-tube synthesis of infective DNAs.
Research upon DNA was long inhibited by the inability of scientists to break the long chains into defined, reproducible segments. In 1970, Hamilton Smith
first characterizes an enzyme, a "restriction endonuclease," that can cleave DNA only at specific nucleotide sequences and thereby reduce a chain to a specific set of fragments. Hundreds of such enzymes, with varied sequence specificities, are subsequently isolated and form an indispensable tool for molecular genetics.
In 1973, Stanley Cohen and Herbert Boyer invent plasmid cloning, thereby making recombinant DNA a practical tool Plasmid cloning permits the amplification a millionfold or more of any specific piece of DNA isolated by the use of restriction enzymes or synthesized in vitro.
In 1977, Fred Sanger describes the first complete sequence of a DNA virus, f X. Knowledge of this complete sequence, 5,386 nucleotides in all, permits the definitive determination of all of the genes of this small virus and provides the first instance of overlapping gene sequences.
15—
Of Honors, Awards, and Prizes
On a May day in 1967, I received with a mixture of surprise, pleasure, and puzzlement a letter informing me that I had been elected a member of the National Academy of Sciences. Surprise because I had not known that I was being considered; pleasure because I was aware, though not very, that the academy was a prestigious organization and membership was considered an accolade; and puzzlement because, although I had been a scientist for over twenty years, I knew almost nothing about this organization, about how one became a member, or even whether I wanted to be one, although now I clearly was, though I had never been asked.
As I subsequently learned, the National Academy of Sciences is an extraordinary organization, unusual in almost even feature. It is a quasi-governmental organization, founded during the Civil War with a congressional charter to make available to the federal government the expertise of the scientific community. Yet it is also, and importantly, a quasi-private organization that receives no direct funding from the government, that elects its own members and officers, and that can initiate studies and projects of its own in addition to those it undertakes on request for the government. Located in Washington, the academy serves in some degree as an intermediary between the intensely political world of the Capitol and the academic, sometimes arcane world of the scientist.
The role of science has changed drastically since the Civil War. Government has expanded and most government agencies have acquired
their own in-house technical expertise. At the same time, government has become the principal patron of science and federal funding is the sine qua non of research in every field. The government uses the academy as a source of external, impartial, expert advice on a host of technical issues arising in modern society. The academy is able to provide this because of the scientific eminence of its limited membership and because of a conscious effort made to establish balanced panels to analyze each issue, followed by scrupulous, thorough review of reports before they are released.
While the academy thus serves an important national function, its procedures and activities are in many ways baroque and it has some major limitations.
I was elected to and served on the Council, the nominal governing body of the academy for three years, 1970 to 1973. The academy also sponsors a major scientific publication, The Proceedings of the National Academy of Sciences, and I served as chairman of its board of editors for eight years, from 1972 to 1980, all of which both permitted and required me to achieve some understanding of the capabilities and limitations of the academy.
A remarkable amount of the time and energy of the academy is devoted to self-perpetuation, to the election of the new members. Only sixty may be elected in any year and while this number has fluctuated somewhat over the years, it has in no way kept pace with the increase in the number of active scientists, so membership has become progressively more exclusive. Since new members are nominated only by present members and are elected by vote of all members, not surprisingly membership tends to be concentrated in a small number of elite research universities—Harvard, Berkeley, MIT, Caltech—and in certain disciplines. The latter effect, which militates against election of members in newer disciplines, is from time to time countered by action of the academy's Council, which can provide for special nominations that bypass some of the lower-level hurdles to election. The former effect, institutional concentration, is never addressed and indeed is considered reasonable and proper.
Though the academy was initially dominated by the physical scientists, in more recent years the biological scientists have achieved nearly equal status. The divisions of the academy representing applied science, the medical sciences, and the social sciences have distinctly lesser influence. In fact, the limited role accorded to the applied sciences in the academy inspired the formation in the 1970s of a parallel sister orga-
nization, the National Academy of Engineering, established by engineers who felt that their profession was not receiving appropriate credit for its national contributions. The two separate academies collaborate in certain responsibilities and coexist in a sometimes uneasy partnership.
In an effort to stave off the formation of yet another academy, the National Academy of Sciences established a somewhat autonomous branch, the Institute of Medicine. The institute is concerned primarily with the sociological, organizational, and policy aspects of medicine as a profession, as distinct from its scientific component, which is left primarily to the academy.
As membership in the academy is a recognition of distinguished scientific contribution, it tends to be a venerable institution, with the average age of election in the fifties. After I was elected, Ray Owen remarked that he particularly enjoyed the academy meeting as one of the few where he could still be among the younger group present. However, while advanced in years, the membership is by no means devoid of spirit, as was evidenced by the sight of several hundred academicians marching in the aisles while the Preservation Hall Jazz Band played "When the Saints Come Marching In" at an evening concert.
While the Council, twelve members elected for staggered three-year terms, is the nominal governing body, the president is the only full-time officer, elected for a six-year term and eligible for reelection. The president has a staff and, as he or she is present before, during, and after the monthly Council meetings, plays a major administrative and policy-making role. During my period of major interaction with the academy, Philip Handler was president. Aristocratic, eloquent, skilled in the ways of Washington politics, he ran the academy in an increasingly personal fashion as his twelve-year term progressed. The membership was by and large satisfied with the arrangement as long as it determined those features in which it had real interest, the disciplinary organization of the academy and the distribution and election of new members.
As one who had lived within the cloistered walls of academe, I at first found the wholly political life of Washington—in which who one knows, and how well one knows them, can be far more important than the logic of an issue—bizarre and uncongenial. As I became more familiar with this scene and realized its imperative, I became more appreciative of the role that Handler and those like him played in meshing the needs of science with the wishes of Congress and the administration.
The academy is able to serve the government well in its basic role as the provider of dispassionate advice on technical issues. The academy
cannot serve the government as provider of advice concerning science policy. Issues such as the allocation of resources among disciplines or the establishment of priorities, which thrust different factions of the academy into competition, cannot be resolved within the academy. Nor has the academy been notably successful in the formulation of policy with respect to those social issues for which the scientific and technological factors are significant but not overriding components. While one might hope for a more rational approach to the resolution of such questions, in practice the intricate political compromises of Washington appear to generate the only acceptable solutions.
As chairman of the board of editors of The Proceedings, I was responsible for maintaining the quality of its articles, assuring its fiscal solvency, and placating occasional irate members. While the content of The Proceedings ought to reflect the diverse interests of the academy membership, in practice the publication tends to be used principally by a selected subset. In recent decades, papers of the biologists—particularly the molecular biologists—have dominated the pages of The Proceedings . Of course, all scientific journals are subject to a specialization of readership and authorship. Authors want to publish in those journals that are read by the prominent workers in their field. Repeated efforts to diversify the scientific fare and stimulate the submission for publication of papers in the physical or social sciences have failed. These disciplines have their own journals and it is in those journals that readers look to learn what is new. A new development in physics published in The Proceedings would not be seen by most physicists because The Proceedings has little of interest to them.
Members have the privilege of submitting self-authored articles to The Proceedings without review. The assumption is that articles from such distinguished scientists will surely be meritorious. Mostly this is true, but occasionally, especially in articles submitted by some of the more senior members, this standard is not met. The chairman of the board of editors has the authority to reject a paper even when submitted by a member. If the paper was outright embarrassing or, say, bore on medical concerns and—given the prestige of the academy—might induce some patients to forsake other more efficacious treatments, I would write to the author to suggest that the paper was not up to his or her usual standard. Outright rejection never proved necessary.
And I will always remember the fine paper submitted by Joel Hildebrandt at the age of ninety-six, together with a handwritten cover letter in a perfect, unfaltering hand.
For the most part, scientists do not become wealthy. In some dis-
ciplines, industrial consulting and entrepreneurial opportunities provide exceptions, and a few have written lucrative textbooks, but in general scientists are far less well remunerated than are other professionals such as physicians and lawyers, not to mention MBAs.
As one consequence, alternative modes of reward—awards and prizes—have been developed. These are primarily honorific, providing only modest financial sums. Such modes of recognition are usually found in the more mature disciplines and are sponsored or dispensed by well-established professional societies. The awards are often named for a distinguished member, usually deceased, of the discipline, and the selections are made by senior members of the society.
In general, these prizes recognize a distinguished career of significant contribution in a particular field and as such are awarded to scientists at the peak of their productivity, or beyond. Some awards are specifically designated for younger members of the profession. Receipt of such an award can have a favorable effect on one's career. In a sense, such an award is a self-fulfilling prophecy, for the recipient is more likely to obtain a better academic position, to attract better students, to receive larger grants, and so on. In science as in other aspects of life, there is a natural tendency for "the rich to get richer." Regrettably, such awards are not usually available in the newer interdisciplinary or ground-breaking fields, which often do not fall within established professional boundaries. Their lack expresses and reinforces the incrementalism and rigidity of the disciplines.
I have been honored by two major awards; both came a few years after the most significant f X discoveries.
In 1968, I received the California Scientist of the Year Award. Unlike most, this is a cross-disciplinary award given to the California scientist in any field whose accomplishments in the previous year are deemed to be of the greatest significance. The award is sponsored by the Museum of Science and Industry and the selection made by a panel of senior California scientists. Luis Alvarez was chairman of the panel that awarded my prize. In addition to the citation, presented at a black-tie banquet attended by distinguished members of the community, a five thousand dollar prize was also awarded. Receipt of this award made me aware of the incidental benefits of such an accolade—increased recognition at my institution, press conferences and publicity, honors that accrue not only to me but also to my family and institution, and even the rediscovery of old friends and acquaintances with whom I had lost contact.
Some years later, as chairman of Caltech's division of biology, I had
the privilege to nominate Roger Sperry for the Scientist of the Year Award, which he received and most eminently deserved.
In 1969, I received the Beijerinck Virology Medal of the Royal Netherlands Academy of Sciences in Amsterdam. Beijerinck was a famous Dutch microbiologist who in the 1890s discovered the virus of tobacco mosaic, which he described as a contagium vivum fluidum —a "contagious, living fluid," so named because he could transmit the disease with his extracts but could see no cells or particles therein with the light microscope, nor could he eliminate the infectivity with any filters he had available.
My studies of f X, one of the smallest known viruses, although quite visible in the electron microscope, had clarified and extended Beijer-inck's concepts at the particulate and molecular levels. The prize consisted of an engraved gold medal and two thousand dollars, which sufficed to bring me and my family to Amsterdam for the award ceremony. It was my privilege to address the Royal Netherlands Academy. As an accompaniment to receipt of the prize, I lectured at each of the five leading universities in the Netherlands—Leiden (founded in 1575), Utrecht, Amsterdam, Nymegen, and Gronigen—and was thus able to meet most of the leading Dutch microbiologists, as well as to see Holland in January.
Prizes are pleasant, but in the science of today such priority is largely an "ego trip" that gives one an illusion of leading the pack instead of merely riding the tide. In fact, nature exists and the discovery is waiting to be found; if scientist A does not find it one year, scientist B will the next. A does so first because, most often unwittingly, he or she has chosen the more accessible instance or has access to the more relevant techniques. The real inventors are those who create and develop new techniques—the ultracentrifuge, gel electrophoresis, the electron microscope, the scintillation counter, and so on. But most often they are not the ones to make the biological applications and thereby receive the acclaim for discovery. Yet without them, molecular biology would not exist.
The most famous prizes are the Nobel Prizes. These differ from most others in several ways They are highly remunerative. They are awarded only in certain fields, designated by Nobel, and are awarded primarily for consequence of a particular discovery, that is, whether the work recognized has proven to be of seminal importance for further development in the field. Sometimes consequence can be quickly recognized, sometimes it can be delayed as long as forty years. The prize is not
awarded, necessarily, for imaginative ingenuity, elegance of experimental design or execution, sustained contribution, or even (always) correctness. As a result, recipients of the prize are not always as yet members of the national academy (an oversight usually quickly corrected).
I have known almost all of the recipients in biology and medicine, and many of those in chemistry, since 1950. In my experience, the recipients of the prizes are good scientists, some very good scientists. But in nearly every instance, one could name half a dozen others of equal ability whose contributions, averaged over their professional lifetimes, would rival those of the recipients. It might be argued that the recipients should be specially rewarded precisely because they had the prescience to work on problems that had great consequence. But in many cases, this could not have been known in advance. In others, it was obvious in advance that a solution to that problem would be of great importance, and the prize winners were those who happened to choose a more favorable system to explore.
Understandably, the prizes tend to recognize those who make dramatic advances but often overlook those whose prior advances and inventions made the "breakthrough" possible. Thus, Gilbert and Sanger deservedly received the Nobel Prize for their development of means to sequence DNA, but no award was given to those who invented and perfected the technique of gel electrophoretic separation that made their work possible. Likewise, several Nobel Prizes have been awarded for research involving "recombinant DNA," but not to Boyer and Cohen who invented this technique.
Nobel Prizes probably served a different function in an earlier era, when scientists worked more as individuals and science was less connected and scientific progress more sporadic. Today, the achievements recognized by Nobel Prizes are mostly only slightly higher crests in the advancing waves of scientific progress.
However, the publicity of the Nobels creates its own aura. Even major institutions feel obliged to pay obeisance to the selections of the Swedish Nobel Prize committees. Each spring at Caltech, as biology chairman, I prepared a schedule of proposed biology faculty salaries for the next year to discuss with the provost. It was understood that any Nobelists would be at the top of the list. Later, all of the division chairpersons would gather with the provost to review all faculty salaries, to seek to achieve rough parity between the divisions, and to recognize any faculty whose contributions may have been split between divisions. In accord with institute tradition, the Nobelists in physics were always
at the top of the institute list and all other salaries were pegged to theirs.
The prize doubtless serves an important public relations function for the scientists and institution concerned and for the image of science to the general public, just as Oscars do for the movies. But within their respective professions, each is viewed very differently.
16—
Transition 4
At some time in my late forties I realized that I had "made it." My research was internationally acclaimed. I was the chairman of the best biology department in the world. I no longer needed to "prove myself" to myself or to others. I had achieved in my research and career what I had set out to achieve so amorphously many years earlier.
In a sense all of that accomplishment was like the uncoiling of a spring that had been formed very early in my life—the realization of values and goals imprinted as a child and focused at MIT. It was as though the program had come to the end of the tape. But where to now? With the imprinted goals achieved, what came next? Some would call this a mid-life crisis, but I had no sense of crisis but rather one of relief and decompression. Long-repressed interests outside of science reemerged.
The tone and ambience of science were changing—the result of its own success. When I entered science, it was in the popular mind an arcane but benign profession. It was peopled largely by scholars who had a driving curiosity to know how the natural world worked, and who could be nothing else but scientists. As a career, science did not promise much material reward but offered great intellectual satisfaction.
The Second World War had convincingly demonstrated the value of science to national security, health, and industry. Government funding then greatly augmented the resources available to science and with them the potential of careers in science. During the 1960s, I observed the entry of a somewhat different type of science student—bright but not
driven—who chose science as an acceptable professional career occupation but who might just as well have become a lawyer, physician, developer. Between 1948 and 1988, the number of Ph.D.s awarded per year in the biosciences increased more than eightfold.
Science could now be performed on a scale commensurate to the problems at hand with a resultant acceleration of progress. And as science and its offspring, technology, became more and more important in the economy, as society was required again and again to adapt to new technological change, and as some of the impacts of the new technologies (e.g., nuclear weapons, pollution, toxic waste, even overpopulation) appeared less than desirable, so the societal ambience of science changed.
Scientific administration proliferated. The allocation of resources for science inevitably became at least partially politicized, and the societal view of science and technology became increasingly critical, whether a backlash to unsought and unwanted change, a response to perceived environmental degradation or military emphasis, or an expression of a latent anti-intellectualism. The public support for science in a society largely ignorant of its content becomes inherently volatile, resting on the public perception of its consequences. Science is still perceived as arcane, but now it is also considered potentially malignant.
These observations led me to an heretical thought: Should there—can there—be limits to inquiry? The very question incites distress and revulsion in a scientist, whose credo must be that knowledge is good and more knowledge better. But the logic of the recombinant DNA controversy forced me to consider the question as dispassionately as possible. Had it ever arisen before—among scientists, that is, not among those committed to religious or ideological dogma? Yes, it had, to nuclear physicists. Frederick Soddy, a colleague of Lord Rutherford's, had written in 1920:
Let us suppose that it became possible to extract the energy which now oozes out, so to speak, from radioactive material over a period of thousands of millions of years, in as short a time as we pleased. From a pound weight of such substance one could get about as much energy as would be obtained by burning 150 tons of coal. How splendid. Or a pound weight could be made to do the work of 150 tons of dynamite. Ah, there's the rub. . . . It is a discovery that conceivably might be made tomorrow in time for its development and perfection, for the use or destruction, let us say, of the next generation, and, which it is pretty certain, will be made by science sooner or later. Surely it will not need this actual demonstration to convince the world that it is doomed if it fools with the achievements of science as it has fooled too long in the past.
And many of the physicists who worked at Los Alamos on the atomic bomb during World War II had deep qualms. As Victor Weisskopf has written: "Many of us hoped that the number of neutrons per fission would be low enough to prevent the making of a bomb. But it wasn't."
To pose the question invites a paradox. How can we know what we would not want to know? The paradox is compounded by the tautology that the most important discoveries are those least expected.
We accept limits on modes of inquiry. We do not experiment on involuntary, human subjects; we seek to minimize suffering in experimental animals (some would ban all animal research); we avoid experiments that might produce irreversible environmental effects. Implicitly, we value human dignity and our sense of responsibility for other life forms and for the planetary environment more than the knowledge that might thus be acquired. Most often, we assume or hope that the knowledge sought can be obtained by other more acceptable means.
But is there any knowledge we would not want to have, regardless of how it was obtained? Knowledge too dangerous, too destabilizing? Our species evolved and survived with the means to cope with the dangers of a world of human scale. At that scale, the species could tolerate the not insignificant level of irrationality that we know to be present in human behavior. But beneath the surface of that human-scale world lie structures and forces of great power, which we have learned to understand and manipulate to human purpose. Could there be elements of these powers that would be mortally dangerous if available to irrational minds? Does nature set traps for unwary species?
If hydrogen bombs could be readily made in a garage workshop, all of human society would be in mortal peril. Happily, it is not that easy. But would we encourage, or allow research to make such simple manufacture possible? Does biology, through recombinant DNA, offer a potential analog? And, if so, could inadvertence, let alone malevolence, unleash disaster?
I was, however, as I am today, most reluctant to concede that humanity should forego knowledge of any kind forever. I therefore thought to introduce another dimension into consideration—time. Discoveries that might be of great potential harm in one era might be innocuous in another. In a truly peaceful world, the discovery of atomic energy might never be applied to weapons. Should then more research and intellect have been devoted to the search for routes to lasting peace and less to nuclear physics?
Is there a preferable sequence for discovery, just as there is a necessary
sequence for the onset of gene expression during the development of an organism? Are there more opportune times for the exploration of certain areas of knowledge? Could we discern such a program and apply it to the allocation of research resources?
These seemed to be meaningful questions, worthy of sustained exploration. These emerging concerns needed to be addressed by minds well informed and perceptive as to likely future developments. But the best minds of science were focused elsewhere. Caltech, MIT, and the national academy were largely ignoring this rising tide, except when it directly affected their specific interests.
17—
Science and Society—
Toward Wider Horizons
We are at a hinge point in evolution. Some two hundred thousand years ago, Homo sapiens emerged on this planet—out of a panoply of life—mostly mute, unself-aware, living, multiplying, dying. We emerged with this body, this frame, these hands, this mind, unaware of any past, ignorant of our inner selves, innocent of the future.
Somehow we found our way. We invented language, writing, agriculture, industry, social organization, and science and only in the last hundred years or so have we begun to learn about the physics and chemistry of our inner selves and consequently about our long ago past; about our intricate machinery and why it can go awry; about the inheritance that makes worms become worms, birds birds, and us humans.
What a wonderful time to be a biologist.
The mysteries fade in the light of knowledge, but with knowledge and understanding can come the urge to intervene, to change, to modify. But the human mind, which is so good at the analysis of what is, falters before what ought to be. This is hardly surprising. Our mind did not evolve to cope with such questions. Evolution has not confronted such issues until now.
Curiously, my conscious reflection on this profound change in the human condition all began with a request to give a Monday Night Lecture. The invitation seemed to tap a previously unconscious, unexpressed vein of interest and concern. By 1965, I had been immersed in science for nearly three decades—a student, a researcher, a teacher, I
had rather singlemindedly pursued a scientific career, interacting with other scientists, teaching future scientists. Now several events were to change my path in the coming decades toward much wider and hazier horizons.
The Monday Night Lectures (now called the Watson Lectures) were a Caltech tradition. On most Monday evenings during the academic year, a Caltech faculty member would attempt to describe his or her research—methods, objectives, results—in terms understandable to an "educated" public. The lectures were one hour, followed by a question-and-answer period. Most made considerable use of visual aids. Over the years, a considerable audience of five hundred to one thousand persons had developed for these lectures, including many of the Caltech faculty. They were a successful means to reduce possible estrangement or tension between the institute and the resident community.
Having never before attempted to present my research program to a nonscientific audience, I took the "challenge" seriously. My title was "The Book of Life." I endeavored to compare the genetic information to the information in a book—like a book of recipes or a manual of flower arranging—written in an unknown language, like a Mayan codex, and I sought to explain how far we had come in deciphering this text and what it meant and what it would mean when the knowledge was more complete. I had made many diagrams for the purpose.
The night of the lecture arrived during one of the torrential downpours Pasadena can have in the winter. I anticipated minuscule attendance, but the audience was loyal and a good crowd appeared. To my pleasure and surprise the lecture was well received. Indeed, the substance was later printed by Addison-Wesley as a "separate" and sold many thousands of copies.
The lecture had another consequence. In the fall of 1966, Caltech was celebrating its seventy-fifth anniversary in Pasadena. A major symposium was planned on the subject of the future of science. Because of my Monday Night Lecture, I was asked to present a lead address on the future of molecular biology and its potential implications. I had never given this larger theme much thought. Scientists are generally immersed in their scientific questions, in daily research and the planning—invention, really—of the next experiment. The larger, more cumulative impacts of scientific knowledge are not often within their ken, and in any case seem rather speculative and remote.
But for the next six months my mind kept returning to these questions and I found I would jot down notes for this talk at all times—
early in the morning, during a car ride, at a concert intermission. It was during this time that I came to realize that the advances continually being made in genetics would before long have the most profound social consequences. I could not foresee the specific pattern of scientific advance that would lead to the potential for genetic intervention. But nature clearly had developed means for genetic modification and recombination and we would surely, in time, and sooner rather than later, unravel these mechanisms and have this capability at our disposal.
We were at an epochal moment, not only for our society or for Homo sapiens but for all of life on earth. For the first time in the long course of evolution, for the first time in all time, a species was coming to understand its origins and its inheritance, and with that knowledge would come the ability to alter its inheritance, to determine its own genetic destiny, as well as that of other living species. Through DNA, biology was moving beyond analysis to synthesis.
This was a transformation without precedent. The issues that would be raised far exceeded the boundaries of our historical morality born of human experience. A few novelists, Aldous Huxley for one, had jousted with the issues, but now fiction could become reality. What principles should guide us? How should decisions be made, how could agreement be reached in a fractured world society? In this light, the links between our social structure and the givens—to date—of human existence became evident. Our size, our life span, our numerical equality of male and female, our genetic diversity and accompanying normal range of talents and traits, all are imbedded in our social order. And all now are potentially mutable.
The potential for intervention in human inheritance raises fundamental questions of the meaning of "equality of opportunity," of responsibility (liability?) when genetic chance is replaced by design, and of human dignity when an individual realizes that his or her traits have been programmed.
The prospects both exhilarated and sobered. I was exhilarated by this triumph of human intellect yet sobered by the need to use wisely the powers thus unleashed. I have been less innocent ever since.
My lecture was entitled "The End of the Beginning" to connote my perception of this turning point in evolution.
Because this message was ultimately minatory, I was apprehensive that it might not be well received by a Caltech audience, but the audience seemed taken by the revelation of the extraordinary significance, new to them, of the recent advances in genetics, and the talk was very
well received. Indeed, Thomas Watson, Jr., then president of IBM and a Caltech trustee, invited me to Bermuda to present the same talk to his IBM administrative retreat. In the succeeding years, I was invited to present numerous talks expanding on this general theme Some of the titles were a bit pretentious—"Darkly Wise and Rudely Great" (from Alexander Pope); "All Men Are Created Equal?"; "The Brain of Pooh: An Essay on the Limits of Mind"; "An Inquiry into Inquiry"; "Humanism and Science"; "The Galilean Imperative"; "Prospects for Future Scientific Developments Ambush or Opportunity."
One of my lectures, "Science and the Quest for Human Values," was at the Pittsburgh Theological Seminary in connection with the 175th anniversary of their founding. While there, I visited their library and was astonished to find a collection of some eight hundred regularly published journals from all over the world. There were only two I had ever seen. Here was a whole universe of scholarship of which I was completely unaware. At Pittsburgh, I began:
In time it will probably be seen as inevitable that science, which set out simply to explore the universe objectively—without the constraints of, indeed orthogonal to, the concerns of value—should have come to test in the harshest way the fabric of our values Today it takes little vision to see that science is ready to pose to man wholly unprecedented questions of the most fundamental character which will of necessity require a reformulation and a deeper understanding of our basic moral principles.
For us in science, it is frankly still surprising to have come from a new direction upon the oldest of questions. Perhaps, as we reflect, this consequence will tell us something about the geometry of fate and the matrix of the human mind.
We are already confronted with grave dilemmas arising from the only partial triumphs of science, which splinter our older values and often expose their expedience and inconsistency. The Malthusian tide of population mocks our belief in the value of individual man, the flood of factual knowledge overwhelms our faith in the immanent value of truth and thrusts us in the feckless role of the sorcerer's apprentice, the disintegration of death undermines our ancient views of the beneficent role of the healer and the worth of human life. Graver questions yet lie ahead as the biological sciences prepare to change the boundary conditions of man.
These talks brought me into contact with a wide variety of people and I began to appreciate how extremely varied were the reactions to my message, how each sought to incorporate it into his or her frame of reference, with resulting fear, awe, distaste, greed, eagerness, resignation, antipathy, consternation, or dismissal Among scientists, the reaction was most often one of exclusion. "Our mission is to acquire
knowledge. How it is used is not my concern." Or one of temporizing: "These issues may arise at some time in the future but they need not concern us now." Or of apprehension that raising such questions now might jeopardize current research funding. These responses reflect in part the narrow specialization of much scientific education and in part the intense dedication required of an active life in science.
Continued reflection raised broader questions in my mind as to the largely self-directed patterns of scientific exploration, mostly government funded. Scientists, understandably, want to pursue their own research inclinations. And surely at the research project level, who should know better what paths are the most promising? Out of this perception has arisen the "peer review" process in which proposals for research funding are evaluated by panels of scientists expert in each field, ranked in order of promise, and funded as far as available resources permit.
But does the process assure on a more macroscopic level that funds are directed toward research on problems most pressing or crucial to society in general? It seemed to me that this result was not obvious—that, for instance, in a world confronting large and unmanageable increases in population, it might be desirable to place more resources into means of fertility control and less into studies of the aging process. Or more funds might be spent on studies of drug addiction, and less on novel devices for military use. Such issues of science policy should not and could not be resolved solely by scientists, but a forum was needed within science for their discussion. None was, or is, available. I presented many of these thoughts in an article entitled "The Presumptions of Science," published in Daedalus . It did not win me many friends in the scientific community.
As a result of these contacts and cogitations, when the recombinant DNA issue arose in the mid-1970s, I came to it from an atypical perspective.
The development of the recombinant DNA technology provided us with the ability to move genes about from one organism to another almost at will. The universality of the genetic code implied that a gene, a tract of DNA, could engender synthesis of the same protein wherever it was placed, provided of course that it was activated in the host cell. This concept provided the potential to use microorganisms as biochemical factories to produce scarce proteins of pharmaceutical value in medicine, such as human insulin, blood-clotting factors, growth hormones, interferons, and so on.
For molecular biologists, this development provided the means to
generate for study large quantities of any gene of interest, known or unknown. And, of course, it provided the means, by introduction of specific genes, for intervention in the developmental and biochemical processes of plants and animals to alter these for human purposes. In short, genetic engineering was here.
Was it possible, however, that these experiments might, by design or inadvertence, produce an organism of undesirable properties, one that could be a danger to man or to the ecosystem? In the long course of evolution, genetic exchange among unrelated species is a great rarity. The evolutionary pattern is a branching tree, not an interlaced network. We would be creating novel genetic combinations never before seen on earth.
Much of this work would be performed, initially at least, on the bacterium E. coli simply because its genetics was so well understood after thirty years of intensive exploration. But our laboratory E. coli strains had originally been derived from strains found living in human intestines. While undoubtedly attenuated, after decades of laboratory existence, might they still retain the ability to colonize the human gut, now bringing with them all manner of exotic gene combinations? A popular form of experiment at the time was the "shotgun" experiment in which quite random fragments of DNA from higher organisms were inserted into E. coli . The genes borne by such fragments were completely unknown. Might some, by accident, be toxic? And, of course, the potential for deliberate insertion of toxic genes, for the purposes of biological warfare or terrorism, was evident.
I was further perturbed by another class of experiments then underway. At this time, tumor viruses from various animal species—birds, cats, baboons—were being mutated, recombined, and so on. At that time, no virus causing tumors in humans had been discovered and it seemed evident that viruses were not a major cause of cancer in man. But viruses were a major cause of cancer in other species and we might "inadvertently" in our experiments generate a human tumor-inducing virus. I was also concerned by the fact that the new forms—viruses, cells, organisms—were living, self-reproducing creatures that, should they be inimical and should they escape from the laboratory to find a suitable ecological niche, could multiply indefinitely. They could not be recalled, nor would it suffice to halt their manufacture. The generation of new living organisms was qualitatively different than the generation of new inanimate objects or chemicals.
Some of these concerns had been raised within the biological com-
munity. A "moratorium" had been suggested for recombinant DNA experiments and a conference to discuss these issues and potential hazards was convened at Asilomar in February 1975. I attended this extraordinary, unprecedented, and unrepeated event. About 120 of the leading molecular biologists attended. The sessions were divided between those in which the most recent scientific developments obtained with the new techniques were discussed with great enthusiasm (what moratorium?), and those in which the conceivable hazards were described and means considered to mitigate them.
Molecular biologists, including myself, trained mostly in biochemistry and biophysics rather than pathology, had largely treated viruses and bacteria as we would any other chemical, taking enough precautions of sterility to accomplish our own experiments but otherwise exposing products to the air, pouring used cultures into the sewers, and so on. Without much data, a wide range of opinions was expressed with regard to the conceivable hazard of various genetic introductions. A discussion by a panel of lawyers informed the audience as to the liabilities to which they might conceivably be subject.
Means for the containment of recombinant organisms within the laboratory were discussed. Physical containment in sterile rooms with air locks was feasible but costly and troublesome. The concept of biological containment, the use of strains of E. coli deliberately weakened so as to reduce their likelihood of survival outside of carefully controlled laboratory conditions, gained favor. Such strains were not available but could plausibly be made within a few months. (This was an erroneous assumption—it proved to be much more difficult to create and work with such strains.)
Clearly, there was far less interest in the discussion of hazards than of new science. Any means to cope with such potential hazards would inevitably hinder research. Opinions ranged from fear of possible epidemic to cavalier statements that if a pathogen should result and a few persons die, it would only cost a few million dollars. In the end, a compromise was reached in which various conceivable experiments would be graded as to their potential hazard. Combinations of physical and biological containment would be proposed, commensurate to the perceived hazard of each experiment. The restrictions would range from minimal on the most innocuous experiments to bans on the most dangerous. The details would be worked out by an NIH committee.
It was all plausible; however, since no one knew the absolute level of hazard, no one was sure just where to "float" this entire matrix of
regulation. To nobody's surprise, it was in fact adjusted so that the experiments that most scientists wanted to do at once could be done with minimal precautions, thus buying time to provide more elaborate and safer facilities for experiments to be done in the future. Science could go forward in good conscience.
In the year or two after Asilomar, I became increasingly uneasy with the safety of the regulatory mechanisms developed. They seemed to me to be token. If the problem was to be taken seriously, quite other approaches would be desirable. Instead of using an organism such as E. coli derived initially from human waste and capable of exchanging genes with other organisms known to reside in the human intestine, a host organism such as a thermophilic bacterium—which could only survive at very high and uncommon temperatures—might be used. Sophisticated, high-containment facilities might be established at ten or twelve centers around the country at which the more hazardous experiments could be performed.
Others shared this view, but few were willing to speak out against the biological establishment. Because my position was secure, I felt an obligation to do so. On almost every occasion that I did, others, often junior faculty, would let me know that they agreed with my position but dared not to speak out. They feared reprisals from more senior and powerful colleagues who controlled funds and advancements.
The controversy over DNA regulations became more intense and, as often regrettably happens, was joined in by less than rational and illinformed nonscientists bent on ideological or political purposes—the Jeremy Rifkins and the religious zealots. This was my first experience with unwanted fellow travelers. It is not always pleasant to be associated, in common cause, with others whose motives one considers suspect or absurd.
Over time, two developments quite changed our perception of the problem. The E. coli strains in laboratory use proved to be considerably more attenuated, less able to compete with indigenous strains outside the laboratory, than might have been supposed. And even more important, the processing of genetic information, of messenger RNA, in higher organisms unexpectedly proved to be much more complex than in bacteria. As a result of the discovery of introns and splicing mechanisms, it became clear that most genes, simply extracted from a higher organism and placed without modification in a bacterium, could not give rise to a functional protein. While complicating genetic engineer-
ing, this discovery greatly reduced concerns about hazards from "shotgun" experiments.
I continue to believe that, with the knowledge available at the time, my concerns over the potential medical and ecological hazards associated with the introduction of the recombinant DNA technologies were fully justified. We lucked out again. But my opposition to the biological establishment in this matter was personally costly. As one of the few opponents who could not be readily dismissed, I became a target for enmity. I think it no accident that since that period I have not been asked to serve on any NAS or NIH committee, although I had previously done so regularly and despite the fact that I have specifically responded to calls by the president of the national academy for its members to become more personally involved. Such is the price of offense to vested interests.
Persistent dissent is treated harshly within the scientific community. Committed to a single truth, scientists tend to dismiss and exclude those who willfully do not "see the light." Empirically based, science is a self-correcting enterprise. There have been few instances in which long-held, seemingly established concepts have been disproved. Thus, the persistent skeptic is viewed as at least eccentric, if not fanatic, and harboring hidden motives.
Throughout this period of engagement with questions of values and issues of controversy, I became increasingly concerned with the perceived failures of our educational system. The bulk of our citizenry is technologically illiterate. We are creating an increasingly technological society in a democracy with a public largely unprepared even to understand the new and complex issues emerging from our enlarged powers. And conversely, the small group of technologically informed citizenry are largely unconcerned with the social implications of their activities. Their education has not prepared them to cope with the vexing questions of social and cultural values. Indeed, many have no doubt deliberate pursued a career in science with its clarity and objectivity so as to escape the complex, ambiguous, sometimes unresolvable questions of public policy.
Scientists are not innately more rigorous or logical, but they face daily the task of comprehending an objective reality. Bias and cant, aesthetic preference and moral conviction are but snares. The natural world is as it is, not as we might wish it or would construct it. Constrained thus to objectivity as a way of life, scientists can only view with
wonder and alarm, even scorn, the self-serving passions that so often pass for argument in most social and political arenas—the hyperbole that contorts logic, the myopia that accompanies self-righteous claims of superiority The limits of evidence are far transcended; the possibility of error seems never admitted.
Scientists, on the whole, wish simply to pursue their investigations of nature—to explore, to discover, to satisfy their curiosity. Nature can be obscure, but nature does not deceive. Yet their investigations are increasingly costly. Because funding is public, the scientifically ignorant public must continually be persuaded that it should, in its best interest, support scientific programs. Thus, the entire enterprise rests on a public confidence that in turn rests more on faith than knowledge. At the policy level, then, the scientific establishment is deeply insecure. It is little wonder that its leadership feels obliged to present a united front and to resist suggestion that any aspects of the scientific endeavor might not be in this society's ultimate best interest.
This state of affairs bears the seeds of future disaster. An ignorant public and a detached yet wary scientific elite could easily be separated by determined political or ideological forces. We have now seen this happen in such diverse areas as nuclear power and animal rights.
By the mid-1970s, it seemed to me that it might be just as important for the future of science for me to devote my efforts to educational reform as to further advance scientific knowledge—to the design of educational patterns producing a more technically literate citizenry and, particularly, a more socially aware scientific cadre.
But where to do this? Caltech, trapped in its own scientific success, dedicated to the most advanced scientific research, was not a promising site for curricular innovation. Nor could the institute be substantially broadened. In the early 1970s, the American Academy of Arts and Sciences was looking for a new academic site in which to establish a center for advanced study in the humanities. I had suggested Caltech. The academy leadership was truly intrigued by this suggestion for possible liaison between a humanities think tank and a small preeminent scientific institution. Conceivably, "two cultures" could produce a dramatically successful synergism.
But the proposal, while not rejected by the Caltech administration, was not pursued with enthusiasm, and the center went to North Carolina instead. A pity!
18—
The Caltech Years—
The Chairman
"I think you mean 'million,' Harold." Harold Brown and I, as the new president of Caltech and its chairman of biology respectively, were going over the biology division's budget for the next year. Until very recently, Brown had been secretary of the air force with a budget of some thirty billion dollars per year. The Caltech budget at that time was about thirty million dollars per year. Since the budget tables provided only the first three or four numbers, omitting the trailing zeroes, his numerical confusion was understandable. After the second correction, I stopped. The zeroes would soon enough correct themselves.
To become president of Caltech or chairman of its division of biology is to join a distinguished roster. Thomas Hunt Morgan became the first chairman of the division of biology at Caltech in 1928. Morgan, who received the Nobel Prize in 1933 for his brilliant studies of inheritance in the fruit fly, drosophila, was the most eminent geneticist of his time. He and his research group had been at Columbia University for more than two decades. That Robert Millikan, Caltech's president, was able to persuade Morgan at the age of sixty-two to uproot from Columbia to a small, little-known institute on the then-remote West Coast surely bespeaks a most persuasive man.
Millikan had raised a million dollars from a neighbor, the industrialist William Kerckhoff, to build the Kerckhoff Biology Laboratory and to endow the new division of biology. Caltech had been created in 1920 out of the existing Throop Institute (a practical trade school for training machinists and nurses) by three distinguished physical scientists, the
astrophysicist George Ellery Hale, the chemist Amos A. Noyes, and the physicist Robert A. Millikan. (Both Hale and Noyes had been associated with MIT.) Biology, which was introduced arbitrarily by Millikan, was a distinct departure from the earlier concentration in physical science and mathematics and is reported to have produced some faculty grumbling: "Biology! Will theology be next?"
Millikan and Noyes foresaw that biological processes would be increasingly amenable to chemical and physical investigation and it was this vision, and the potential for its realization at Caltech, that was persuasive to Morgan. It is curious, however, that the first Kerckhoff biology building did not have a single fume hood for chemical research.
Millikan was peculiarly astute in his selection of a geneticist to head the new division. Genetics was destined to become the central theme of biological research in the twentieth century (and likely beyond), but this was not obvious in the 1920s when experimental embryology and physiological biochemistry were in their heyday. Morgan brought his drosophila group with him, including Henry Sturtevant, Calvin Bridges, and Sterling Emerson. California was then considered so remote that these faculty were assured they could return every other summer to the marine laboratory at Woods Hole, expense paid, to maintain their scientific contacts.
After Morgan's death in 1943, the chairmanship was held by temporary appointments until Lee DuBridge brought George Beadle, who received the Nobel Prize in 1957 for his studies of biochemically deficient mutants in the mold neurospora, from Stanford. Under Beadle, the division began slowly to grow and diversify. Notable arrivals included Delbrück in 1948, Ray Owen, a geneticist interested in immunology, in 1946, and the neurobiologist Roger Sperry in 1954. Beadle left in 1960 to become president of the University of Chicago and was succeeded by Ray Owen. When Owen decided to step down in 1968, I was asked by DuBridge to succeed him.
In 1968, after twenty-two years as president of the institute, Lee DuBridge was approaching retirement. He had been an outstanding administrator. In his early years, he revitalized the institute. His continuing good judgment in science had retained the confidence of a somewhat arrogant faculty; his warm manner, his ardent conviction of the value of science, and his exceptional ability to present the aims of science in popular terms won broad local and national support for the institute. While accepting of specific tasks, he, unlike many of his confreres, had
eschewed long-term commitments to the Washington scene, believing that Caltech required his primary attention and energies.
In his later years, the success of his leadership in turn led to a gradual growth of institute activities, which outgrew his capacity for direct supervision. His personal style of administration did not permit him to develop an appropriate administrative substructure. Thus, toward the end of his tenure, the institute was in some disarray as regards finance and administration.
As a private institution, Caltech is formally governed by a self-perpetuating board of trustees. Their most important function is the periodic selection of a president. Having done this, a wise board—and Caltech has a wise board—leaves the academic administration of the campus to the person they have chosen. They continue to keep themselves informed, they maintain a close watch on the financial status of the institution, and they serve as a source of and conduit to the major gifts essential to the survival of all private schools.
In looking for a successor to DuBridge, the trustees were evidently seeking an individual with scientific training who also had the extensive managerial experience then needed to realign the institute's finances and administration. Good contacts in Washington, the primary source of research funds, were also desirable. The two leading candidates were James Fletcher, who had been director of NASA, and Harold Brown, former director of Livermore National Laboratory and the current secretary of the air force. After the election of Richard Nixon in 1968 and the onset of a new Republican administration, options changed quickly. DuBridge was asked by Nixon to become his science advisor, and Harold Brown obviously needed a new position and became instantly available.
His appointment as science advisor seemed a most fitting cap to DuBridge's distinguished career In fact, it developed otherwise. Unable to cope with the hardball, partisan tactics of the Haldeman-Ehrlichman shield around Nixon, DuBridge retired after two years.
Because of his military connections, Harold Brown's candidacy for president was regarded with some suspicion by Caltech faculty. An intensive series of meetings by Brown with various faculty groups allayed most doubts. With the urgency generated by the immediacy of DuBridge's departure, the trustees quickly selected Harold Brown to be the third Caltech president.
He proved to be an excellent administrator with rather little educa-
tional vision. He was content to let the faculty develop their own concepts of desirable research and educational directions. At an institution of the calibre of Caltech, this policy works for a time. Even at Caltech, however, faculty tend to reproduce themselves and their programs and a guiding, innovating hand is occasionally needed. Unquestionably brilliant, Brown lacked the personal warmth of DuBridge and never achieved similar personal rapport with faculty or supporters.
As president, Brown chaired the Institute Administrative Council (IAC). Because Caltech is small, the chairmen of each of the six disciplinary divisions of necessity also play a major role in the relatively small central administration. All major policy questions are discussed in the IAC, composed of the president, the provost, the six chairmen, and the vice-presidents for finance and for development. Thus, a division chairman became well acquainted with issues of institutional policy as well as with the concerns of his own division. Chairmen differed widely in their abilities to adopt an institutional point of view as distinct from the parochial interests of their own constituencies.
Under Lee DuBridge's more autocratic style, the IAC meetings were not so much forums for discussion as conventions to confirm decisions previously made in his office. Harold Brown had developed a different style while secretary of the air force in dealing with military commanders. Under his leadership, meetings of the IAC were much more vigorous, although the final decisions were clearly his to make. Men of eminence in their respective fields, these were no "shrinking violets," and discussion was intense, albeit respectful. Jack Roberts in chemistry was especially vociferous in defense of his views. Bob Huttenback, later to become chancellor at the University of California, Santa Barbara, was an effective advocate for an expanded role for the social sciences at Caltech. The meetings were frequently enlivened by differences between Robert Christy, the provost, and William Corcoran, the vice-president for development. Christy, a physicist, was direct and tended to see issues in black and white. Corcoran, a chemical engineer by profession, spoke in a very prolix manner, spiraling about his subject in ever narrower circles until he was finally ready to make his point. His perceptions were usually complex, even baroque. The two distinct visions and styles often clashed, producing sparks.
The IAC was concerned with academic, fiscal, and administrative issues. The problems confronting the institute in that period were significant but not critical. All tenure decisions in all disciplines were discussed there. The academic-research programs of the divisions were
reviewed. Those in biology, chemistry, earth sciences, and astronomy were considered eminent and in good hands, with sufficient opportunities for renewal with young appointments. Physics, especially theoretical physics with both Richard Feynman and Murray Gell-Mann, was outstanding; the lack of significant activity in solid-state physics did not seem troubling.
The mathematics program, however, was not considered to be of a stature commensurate with other facets of the institute and several efforts were made, unsuccessfully, to recruit leading mathematicians. An "ideological" spilt between the "pure" and "applied" mathematicians further complicated this area.
Engineering at the institute was a more severe problem. Student interest in engineering had ebbed to a low of 25 percent of the student body. While there were several outstanding faculty in various fields, it was widely felt that the institute had "missed the boat" in the development of computers and the associated technologies, although to some extent this was compensated by the activities at the Jet Propulsion Laboratory. The engineering faculty was aging, but there were few retirements and therefore limited opportunities for faculty renewal. Successive chairmen wrestled with this problem with limited success in this period.
Modest efforts were also made to broaden the institute's offerings in the social sciences, particularly in the more quantitative areas of economics and organization theory.
The IAC considered other, more immediate, often vexing issues of overall institute policy, including the provision, in a financially feasible manner, of adequate computing capability for the campus. Sharing facilities with JPL proved to be a salutary solution, though campus-JPL relations in general required continuing attention. The issue of classified research at JPL, undertaken at specific government request, was troubling especially during the Vietnam period of unrest. The institute received a modest but not insignificant "management fee" for its operation of JPL. The insistent temptation to build this into the institute budget had to be resisted so as not to make the institute beholden to continuation of this relationship, should that become undesirable. Periodic changes in "overhead" provisions on government grants and contracts produced corresponding fluctuations in the institute budgets and funding for graduate student support, which had to be met with one or another stratagem.
The institute's astronomy program, together with the astronomy di-
vision of the Carnegie Institute of Washington, operated the Palomar Observatory (which belonged to Caltech) and the Mt. Wilson Observatory (which belonged to Carnegie) jointly as a consortium. This arrangement worked for some years with a manageable degree of friction. The usefulness of Mt. Wilson, however, was increasingly compromised by light pollution from Los Angeles. The Carnegie Institute determined to shut down Mt. Wilson and to build a new observatory in Chile. It desired Caltech's financial participation in this project; however, at that time Caltech did not see such investment as a high priority. The consortium subsequently dissolved and Carnegie went ahead on its own with the Southern Hemisphere telescope.
For much of its history, Caltech had been an all-male institution. Women graduate students were first admitted in 1954 and women undergraduates in 1970. The proportion of undergraduate women quickly rose to 15 to 18 percent. At this level, however, their limited numbers created problems of mutual support and social ambience. The high entrance requirements and the restricted range of curricular options limit the potential pool of women applicants. In recent years, determined efforts have raised the proportion to over 30 percent, a more viable ratio.
This IAC experience with institutional problems in higher education was an invaluable, if only partial, preparation for what was to come.
As chairman, I was expected to attend the annual fall trustees' meeting, which was held off campus, usually at a ranch near Palm Springs. The Caltech trustees are a remarkable group, involving many distinguished citizens, including the chief executives of several of the most technologically innovative companies. While the trustees are concentrated in California, a deliberate effort has been made to include trustees from all parts of the U.S. and from a wide range of activities and industries. They included such civic and industrial leaders as Judge Shirley Hufstedler, later secretary of education; Robert McNamara, former secretary of defense; Thomas Watson, Jr., president of IBM; Arnold Beckman, president of Beckman Instruments; Simon Ramo of Thompson-Ramo-Woolridge; and so on. In addition to providing a broad range of expertise, this composition also gave access to a wide field of potential supporters.
As leading executives, the trustees are very often strong personalities. Brown, having coped with top military brass, was able by sheer intellectual prowess to manage and direct trustee meetings toward his desired ends. I watched with admiration as he verbally sparred with and
subdued a rambunctious Fred Hartley, the irascible head of Union Oil, or corralled an outspoken Howard Keck, the self-made, blunt-speaking oil magnate.
These kinds of issues and the accompanying milieu were distinct from those to which I had become accustomed in research and teaching. Why did I want to become chairman? The responsibilities, while not excessive, would cut into the time available for research, editorial, and other professional roles. Also, I was assuming a major implicit responsibility for the future welfare of the division and my colleagues. Partly, I felt honored that my colleagues, and the president, would entrust me with this role, to follow such distinguished predecessors. Partly, I was curious about the practice of university administration. Partly, I realized that a number of the biology faculty would be retiring in the next decade. The choice of their successors would be critical to the quality and direction of the division. I felt ready and qualified to provide this leadership. And, of course, if I did not accept this post, who would be the next chairman, and would I be satisfied under his leadership?
As chairman, I soon found that administration required a quite different pattern of cognitive skills. Experimental research requires an intense, almost single-minded focus on the question at hand. One lives with the problem, seeks for alternative explanations of the data, searches for a coherent generalization that can be tested. Administration, however, requires instant shifts of attention and quick shuttles from one memory bank to another as different issues are brought for one's consideration. One's thought is more reactive, less self-generated. Indeed, it is rare to find time to think extensively about any one issue. At first, this transition was quite difficult for me. Once it was made, however, going back to the previous pattern was even more difficult.
As a professor, I had shared the usual faculty perception of university administration: an unfortunate evil, seemingly necessary to provide an interface with the outside world, often dedicated more to its own interests than to those of the faculty. A good administration was one that intervened minimally with the wisdom of the faculty. I soon learned to recognize the myopia of that view. The administration does buffer and safeguard the faculty from the external world, but just as much, it protects the faculty from its own rampant self-centeredness. By maintaining civil and orderly processes, it forestalls entropy and preserves the faculty from drifting into anarchy. Even at Caltech, difficult decisions must be made concerning the allocation of resources, the distribution of emphases, and the boundaries of propriety. Wise leadership must seek to
discover the directions of the future amidst the importunities of today.
At its external face, the administration must raise funds, comply with government regulations, cope with lawsuits, and seek to maintain good public and community relations within a society that has little real understanding of the institution's activities or fundamental purposes.
As chairman, I participated in biennial joint meetings of the Caltech and MIT administrations. At these meetings, each discussed the issues it was facing, many of which were, of course, common, such as interactions with the federal government, overhead charges, relations with industry, policies regarding entrepreneurial faculty, patent policies, curricular issues, recruitment of students (especially women and minorities), and fiscal policies, and the often distinctive ways in which they sought to address these. It was most interesting to me, as a former student, to see this other, administrative side of MIT. Much larger than Caltech, more hierarchical, more bureaucratized, more involved with government and industry, the formal MIT style and the personal Caltech style were quite different, although both shared the same problem-solving approach and often, by different routes, came to similar conclusions.
Because of its greater diversity, its closer ties with the military, and its sheer size (which made it easier to gather a critical mass of activists), MIT was not spared the student turmoil of the 1960s. The administrators' accounts of their difficulties, and the efforts needed to cope with the issues, made us grateful for our more benign circumstances.
To be chairman of the biology division at Caltech is to occupy a position of prestige and wide notice. One is quickly asked to perform a wide variety of national services. In 1970, I was elected to the Council of the National Academy of Sciences for a three-year term. In the same year, I was elected president of the Biophysical Society. In 1971, I was appointed to the advisory committee to the director of the National Institutes of Health for a three-year term. In 1972, I became a member of the scientific advisory board to the Jane Coffin Childs Scholarship Fund for a four-year term (which was later renewed). I served for four years on the advisory board to the Scripps Institute and for four years on the scientific advisory board of the Merck Pharmaceutical Corporation.
As almost all of these activities were nonpaid, in effect Caltech subsidized these contributions of my time. Requests for such outside service can become excessive. I had to decline some, such as service as an alumni member of the MIT Corporation, because I was already making
two trips per month to the East Coast. Obviously, my research, my teaching, the biology division, and Caltech itself required some attention.
It is important for the chairman to be alert to the advances and developments in biology broadly so that he can provide leadership and guidance to the division and the central administration when it becomes necessary to replace renting faculty or when the opportunity arises to add new faculty positions. To accomplish this, I read widely; attended diversified meetings, such as those of the American Association for the Advancement of Science, or specialized meetings, such as those of the New York Academy of Sciences, that I otherwise would likely have passed over; and, when visiting other universities to lecture, made it a point to learn broadly about their programs and plans. My activities at the national academy (especially the editorship of the Proceedings ) and at NIH helped to inform me of the latest progress in varied fields.
In the early 1970s, it was becoming clear that the great discoveries of the 1950s and 1960s in molecular biology, which were largely achieved through studies of simple microorganisms, could soon be applied to the study of the much more complex cells and processes of higher organisms. The development of recombinant DNA technology and cloning methods in the early 1970s solved the long-standing difficulty of obtaining an adequate amount for analysis of any one gene of a higher organism. Newer methods of light microscopy and major refinements of the methods of electron microscopy provided the potential for new insights into cellular substructures.
Over the years, I encouraged the division to bring in new faculty who would exploit these opportunities. We added Elias Lazarides, an expert in the area of dynamic cellular ultrastructure, a field that has steadily grown in importance in unlocking the mysteries of cell shape and movement and the varying locations of cellular organelles. The complex field of immunology also seemed ripe for attack by molecular methods. We brought back Leroy Hood, a former Caltech graduate destined to make major advances both in immunology and in the associated area of biological instrumentation.
Giuseppe Attardi set out to unravel the functions of the mitochondria, small bodies present in every higher cell that, curiously, carry their own small pieces of genetic material. He succeeded in completely mapping and deciphering all of the genes of this organelle.
As refinement of technique made it possible for electron microscopy to approach its inherent potential, it became a tool of increasing im-
portance in many areas of biology. We were fortunate to bring on board Jean-Paul Revel, who established a research program and up-to-date laboratory in this field.
Developmental biology had a long tradition at Caltech, beginning of course with Thomas Hunt Morgan. After the sudden and untimely death of Albert Tyler, a student of Morgan's, we brought in Eric Davidson, who continued to exploit the classical system of sea urchin egg development. With Eric came Roy Britten, formerly of the Carnegie Institute, and together they began to analyze developmental processes at the genetic and transcriptional levels.
Subsequently, understanding of many of the genes involved in development and their roles in its early stages has advanced rapidly. Progress has been built in large part on the genetic analysis of development in drosophila, which had been painstakingly worked out at Caltech over many years by Ed Lewis. When the molecular techniques finally became available, Lewis could provide a wealth of genetic information together with the essential mutants and specialized breeding stocks.
My principal accomplishment as chairman was the establishment of a significant program in neurobiology. Caltech had had for many years a low-key program in neurophysiology with Professors Wiersma and Van Harreveld, and it had a singular program in psychobiology with Roger Sperry. I was convinced that neurobiology would be the next great frontier of biology. Armed with the techniques of molecular biology on the one hand and with the techniques of neural system analysis derivable from the field of computer design on the other, great progress seemed possible.
Dramatic progress in one field often derives from the introduction of new concepts developed in another. The availability, of heuristic conceptual models can be a key requirement. Thus, understanding of the mammalian circulatory system relied on the prior knowledge of pumps and pipes. Similarly, development of computers and computer programs that could simulate at least some mental processes has provided useful conceptual models for events in the central nervous system. But the brain is not simply an intricate computer. An elaborate chemistry, genetically programmed, is required for the formation of its complex circuits and, as well, for its continuing function. In the past few decades, a wide and growing variety of neurotransmitters, modulators, and associated receptors has been discovered, all required for the effective and specific transmission of impulses between neurons.
We needed first a leader for the program. Superficially, Roger Sperry
would have seemed the obvious candidate; his experiments on the specific programming of neural connections and on the distinctive functions of the two hemispheres of the human brain were extraordinarily brilliant in design and execution. Indeed, the latter, by demonstrating the existence of two separate consciousnesses in the separated hemispheres, provided one of the few true experiments on the nature of consciousness. But Roger had, unfortunately, the wrong personality, for this role. A loner in research, his opinion of virtually all other neuroscientists was dim at best. His scale of approval ranged from minus infinity, to zero. A "neutral" estimate was in fact high praise from Roger.
Of course, much is known about the outcome of brain function through simple observation, retrospection, and psychological research. What is sorely needed is understanding of the mechanisms at the chemical, cellular, and systems levels underlying these observations. For this reason, I sought to find a leader who could combine a firm grasp of neurobiology with a deep knowledge of the psychological phenomena to be explained. I found him in the person of James Olds.
Olds was at Michigan and was famed for his discovery of "pleasure centers" in rats, regions of the brain for which electrical stimulation was reinforcing. Rats, given the opportunity to stimulate their own brains in these regions, were swiftly addicted to the stimulus to the degree that they would cease to eat or drink so as to continue the stimulation. The neurochemistry and neurophysiology of these regions and their connections to behavioral patterns were clearly of great interest. Olds was also interested in the processing of sensory information and in particular the conditioned filtering of incoming data by centers in sensory pathways to permit the selection of those inputs previously determined to be significant or desirable.
To establish the program, we next needed a new laboratory, building. Happily, Arnold Beckman, then chairman of the Caltech board of trustees, quickly perceived the potential of this new frontier for biology and agreed to underwrite the cost personally. Caltech has repeatedly been most fortunate in the foresight and generosity of Arnold and Mabel Beckman.
With the prospect of a new building and funds for a broad new program, I was able to entice Jim Olds to come to Caltech. He quickly built up a strong group of young faculty members interested in varied aspects of neurobiology. Jack Pettigrew was interested in the role of early experience in the establishment of neuronal connections in the cat. John Allman, building on the work of David Hubel and Torsten
Wiesel, demonstrated the existence in the primate brain of multiple representations of the visual field, each specialized for a particular mode of analysis. David Van Essen explored in particular the pathways and domains revolved in color perception. Jim Hudspeth studied the exquisitely sensitive manner of sound transduction into neural impulse in the cochlea. Mark Konishi sought to analyze the combined roles of inheritance and early experience in the development of bird song in various species. And Seymour Benzer elegantly undertook to identify and characterize mutants affecting neuronal function in drosophila and thus provide probes for more detailed analysis of central nervous system organization in that organism.
Tragically, Jim Olds died in a drowning accident a few years later. While the program suffered from this loss, it has recovered well and continued to develop along the directions originally foreseen.
The new Beckman Laboratory of Behavioral Biology looked across a grassy mall to Baxter Hall, the locale of the division of humanities and social sciences. The humanities and social sciences had long been peripheral to Caltech, considered a necessary part of a rounded (or at least elliptical) education, but not intellectually linked to the institute's primary thrust. I thought that such a connection might now be made between behavioral biology and the humanities and social sciences through the introduction of a program in cognitive psychology. I felt this link would strengthen both sides of the mall and would provide a new coherence to the entire institute curriculum.
I broached this concept to Harold Brown and to the entire Institute Administrative Council. The chairmen of all of the science and the engineering divisions were strongly opposed. They clearly saw such a new division as a competitor for institute resources and one that would provide scant benefit to their programs. Harold Brown took a neutral stance. The power of inertia became startlingly clear. Each of the extant divisions had a strong spokesman, while a new, unborn division had none. The necessity for strong, visionary leadership also became very clear.
As chairman, I had my first experience with what I found to be the most frustrating and unpleasant of my administrative duties—coping with personnel problems. The excellent long-time executive assistant to the chairman of biology had retired a few months prior to my appointment and Ray Owen had hired a replacement. Owen warned me that he was not sure how the new man would work out. He didn't. For several months, I tried to work with him, to instruct him as to what was
needed and how it should be done, but to no avail, and in the end I had to bluntly fire him.
I also had my first, but not my last, experience in coping with alcoholism. A good friend and fine scientist on the biology faculty fell victim to this addiction. Therapists and clinics were to no avail and in the end he had to be coerced into resigning, for he was simply unable to perform his duties. This was a tragedy for himself, his family, and his friends.
But the most unpleasant encounters concerned "negative tenure" decisions, when the biology faculty, had concluded that a young faculty member's performance did not merit the award of a lifetime appointment of tenure. These junior faculty had typically been with the division for six years and had naturally been treated as colleagues. As chairman, I met regularly with individual young faculty members to review their performances, to ensure that they were receiving adequate resources, and to give them counsel. In each of the negative tenure decisions, I had seen the warning signs well in advance and attempted to provide constructive advice. Such advice was rarely taken and the decision, which I conveyed, invariably came as a bitter shock. The angry, disappointed candidates were given another year's appointment during which they could seek—and, at that time, always found—another position, but it was always a year of tension. As chairman, I could persuade myself that the decision was best both for the institute and for the individual, who could find a more appropriate position elsewhere—but that intellectual theorem did not much dilute my emotional distress.
By the mid-1970s, the research with f X was drawing to a natural end. The essential features of the viral structure were known. All of the stages of viral replication had been outlined, the viral genes had been mapped, and their functions deduced. Sanger was elaborating the complete nucleotide sequence of the viral DNA. Kornberg was using f X to disentangle the complex enzymology of DNA replication. Many details were still obscure, such as the manner and order of assembly of the progeny virus particles and the enzymology of lysis of the host cells. But these seemed likely to be of parochial interest, specific to this not especially important virus. Graduate students, perceptive of future scientific opportunities, were choosing to work in other laboratories.
It was time to initiate another research program. A major shift, as into neurobiology, was alluring but would require a few years to learn quite different techniques, acquire a background in the field, and establish a quite different laboratory. I had now taken on a variety of outside interests and commitments that would severely conflict with the
concentrated effort required to establish a wholly new research program. And I believed these interests merited a significant share of my time. After some thought, I therefore decided on a research direction that would make good use of my current skills and established laboratory. At this stage, I also sought a problem closer to practical application for societal benefit. I chose the field of nitrogen fixation.
All living forms require nitrogen, usually as ammonium. The nitrogen comes either from consumption of other organisms or their decay products or, for plants and many microorganisms, by fixation of nitrogen from the atmosphere. Plants cannot fix atmospheric nitrogen themselves but rely on microorganisms in the soil with which they establish a symbiotic relationship. The nitrogen thus supplied is frequently limiting for growth. Crop yields are markedly improved by the application of costly nitrogen fertilizer, produced by industrial processes but coming ultimately from the atmosphere.
The biochemistry and genetics of nitrogen fixation by those microorganisms with that capacity was poorly known and seemed a ripe subject for the application of molecular biology. And the possible benefits to agriculture of improvement in nitrogen fixation techniques were evident. Because this research would primarily involve microorganisms, many of our well-established techniques and laboratory facilities would be immediately applicable, though new modes of assay and equipment for work under anaerobic conditions would have to be added.
Considerable thought was given to the design of experiments to approach this problem. The first steps—acquisition of appropriate bacterial strains and familiarization with assay techniques—were underway when my career took another distinct turn.
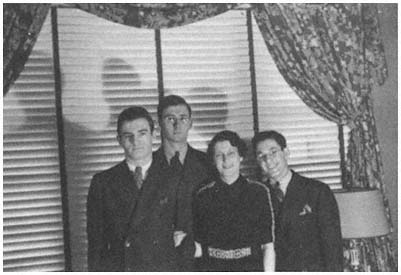
1. With mother, Rose, and brothers, Allen, Jr. (second from left), and
Richard (right), 1938.
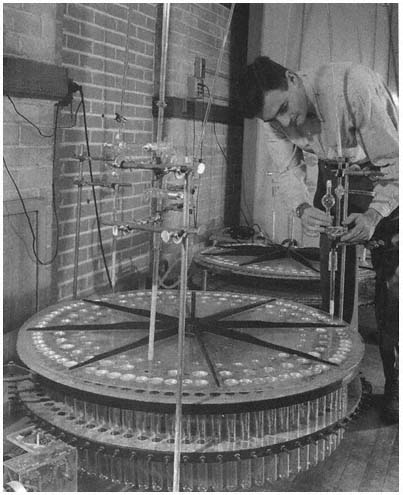
2. Laboratory at Iowa State, 1951.
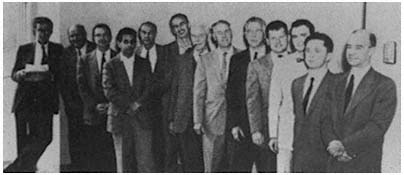
3. Biology faculty and research associates at Caltech, 1958. Left to right:
Max Delbrück, Ernest Anderson, Norman Horowitz, George Laties,
Roger Sperry, Harry Rubin, Alfred H. Sturtevant, George Beadle, Sterling
Emerson, Henry Hellmers, Robert Sinsheimer, Edward Lewis, Geoffrey
Keighley. Absent: James Bonner, Henry Borsook, Herschel Mitchell.
(Courtesy California Institute of Technology)
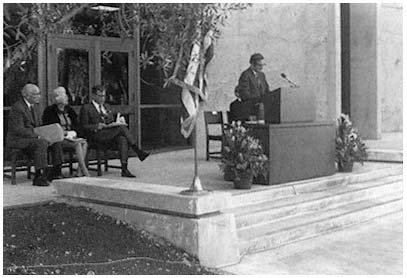
4. Speaking at dedication of Arnold and Mabel Beckman Laboratory of
Behavioral Biology, 1974. Seated, left to right: Arnold Beckman, Mabel
Beckman, Harold Brown. (Courtesy California Institute of Technology)
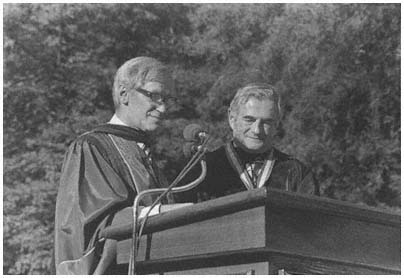
5. Inauguration as chancellor of UC Santa Cruz by UC president David
Saxon, 1978. Photo by B. Lee. (Courtesy UC Santa Cruz Photo Services)
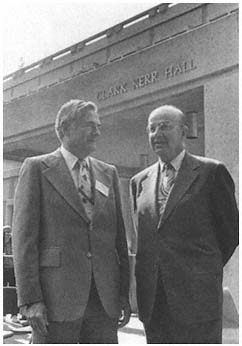
6. With President Emeritus Clark Kerr
at dedication of Clark Kerr Hall on Santa
Cruz campus, 1978.
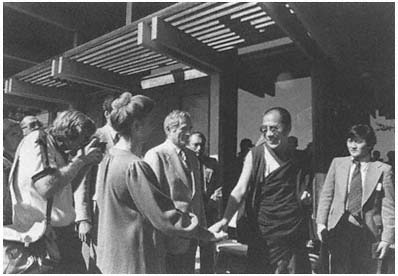
7. With Dalai Lama at University House, UC Santa Cruz. Photo by Carol A.
Foote. (Courtesy UC Santa Cruz Photo Services)
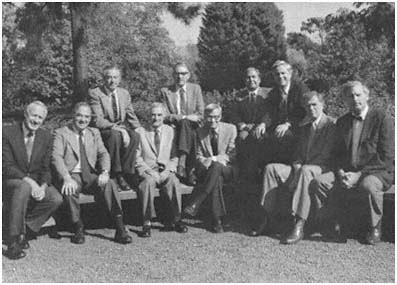
8. President Saxon and the chancellors, 1982. Left to right: Daniel Aldrich, Robert
Huttenback, Julius Krevans, Robert Sinsheimer, James Meyer,
David Saxon, Tomas Rivera, Richard Atkinson, Charles Young, Ira
Michael Heyman. Photo by Saxon Donnelly.
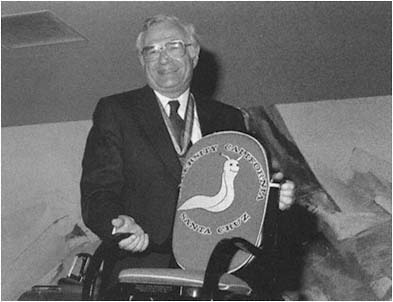
9. At retirement dinner, receiving "chair" symbolic of donated Sinsheimer
professorial chair in molecular biology, 1987. Photo by Don Fukuda.
(Courtesy UC Santa Cruz Photo Services)
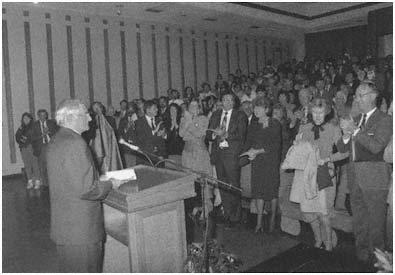
10. Speaking at dedication of Sinsheimer Laboratory, 1990. Standing, front
row, left to right: David Gardner, Libby Gardner, Karen Sinsheimer,
Cliff Poodry, Kathy Stevens. (Courtesy UC Santa Cruz Photo Services)
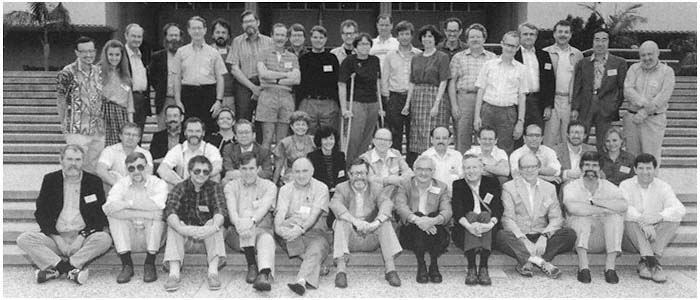
11. At seventieth birthday symposium, gathering of former students and postdoctoral fellows, UC
Santa Barbara, 1990. Front row, left to right: John Abelson, Graham Darby, Arnie Levine,
Larry Dumas, John Newbold, Walter Fiers, Bob Sinsheimer, Bob Nutter, Paul Johnson,
Lee Compton, Lloyd Smith. Center: Ali Szalay, Jim Strauss, Marshall Edgell, Ellen Strauss,
Tohru Komano, Nicole Truffaut, Jane Cramer Harris, Jim Koerner, Moises Eisenberg, Dave Denhardt,
Tony Zuccarelli, Bob Rohwer, Galina Moller. Back: John Hall, Erica Wickstrom, Harry Beevers,
John Sedat, Phil Hanawalt, Harry Noller, Björn Lindquist, Ted Young, Clyde Hutchison,
Nigel Godson, Stan Krane, Alice Burton, Peter Baas, Regis Kelly, Anne Haywood, Lorrie Greenlee, John Kiger,
Helmut Steiger, Karel Grohmann, Barklie Clements, Akio Fukuda, Jean Paul Revel.
19—
Transition 5
"David Saxon is calling you from the president's office at the University of California."
David Saxon, a physicist, had been a classmate at MIT. I particularly remembered him in the atomic physics course. I had encountered him at UCLA occasionally in the intervening years and had noted with interest his selection as president of the University of California in 1975. Why was David calling me on a rainy February. morning in 1977? Simply put, he was looking for a chancellor to head the University of California campus at Santa Cruz, the newest campus in the UC system, and my name had been put forward. Would I be willing to consider this position, to meet on the campus with the search committee and then with himself and other top university officials? I would think about it.
I knew a little about Santa Cruz. My daughter Kathy had been a student there for three years, 1968 to 71, and I had visited her on several occasions. It was an "experimental" campus, distinctive in the UC system for its collegiate structure. It sought to relieve the anonymity and alienation of students on the large UC campuses by clustering them into living and teaching units, the colleges, each with some six to eight hundred undergraduates. Physically, it was extraordinarily beautiful, located in a redwood forest at the edge of meadows overlooking Monterey Bay, seventy-five miles south of San Francisco.
The invitation created an intense personal dilemma. 1 was completing my twentieth year at Caltech—and I loved the institute, its scientific intensity, its freedom from bureaucracy, the wealth of able colleagues
in all of science. I had long since decided I would not trouble to consider offers from other academic institutions. But, in terms of career, I was at a moment of transition. My f X research was phasing out and I as preparing for a quite different research program in nitrogen fixation. I was in my ninth year as chairman of biology and I had informed the president that I felt my tenth year should be the last. I wanted to devote more time to research and scholarship and a fresh chairman with fresh ideas would be salutary for the division.
In addition, I had become increasingly concerned with the dramatic potential and inevitable social consequences of the advances in biology. My experience in the "recombinant DNA" controversy and my inability to move Caltech even modestly toward a broader intellectual base led me to believe that the impetus to consider seriously such issues would not come from within science. Perhaps a new and (as I thought) growing, yet unshaped, university such as Santa Cruz would provide the medium in which my concerns could find expression.
At fifty-seven, I could make but one more major career move if I so desired So, yes, I would visit Santa Cruz, with no commitment asked nor given.
The campus was as beautiful as ever, with sunlit paths, shady ravines, and towering redwoods looming against the sky. But the physical plant, only 12 years old, looked a bit shabby—walkways were crumbling, smudged or scraped walls needed paint. The campus was clearly troubled. The founding chancellor, Dean McHenry, had retired in 1974. His successor, a relatively young professor from Berkeley, had proven inadequate to the complexities of Santa Cruz and had been forced to resign after a year and a half. The present chancellor, Angus Taylor, was a UC veteran sent down from the central administration to fill in until a new chancellor could be selected. At that time, the causes of the disarray and discontent seemed to me either petty or obscure. They seemed manageable. As I was later to learn, however, the pettiness concealed deep ideological differences and the obscurity derived from basic images and policies deeply embedded in the University of California, but then unknown to me.
I met with the chancellor, faculty, staff, and, somewhat to my surprise, students. Their questions were not very probing. All seemed to recognize that the present situation was untenable, but there was no clear vision of the future. They were looking for a savior to lead them—acceptably—out of their sea of troubles. The most prescient question came from an intermediate level administrator who asked: "You have
clearly been a very successful scholar. Why do you want to become an administrator?" I did not realize then that, in the UC system, the two were incompatible. I went on to meet with David Saxon and some of his central UC administration. David indicated his full support for Santa Cruz. In retrospect, I don't think he had a grasp of the depth of its problems.
I returned to Caltech uncertain whether I would accept were the position offered. A week later, it was. I was their first choice.
My career had encompassed the two finest institutes of technology. in the nation, as well as a period at one of its finest agricultural schools. Now I was asked to take charge of a relatively new and (I thought) growing university to guide its destiny into maturation. Little did I know that it was in fact moribund and regarded by many as a misbegotten child of the UC system in dire need of resuscitation. What tipped the balance in my mind? I believe it was the challenge and, as I then thought, the opportunity. I could stay on at Caltech, comfortably and happily, another dozen years in a predictable milieu. Or I could essay a very different role in a very different environment, with a different context and horizons.
I accepted. And indeed it turned out to be different—from my expectations, from past experience—and a tremendous learning opportunity.