Light
Of all the environmental factors, light is perhaps the most readily isolated of all, for its momentary influence on temperature is small and therefore of small importance in influencing circulation or density of the water. Its direct effect on chemical reactions in the sea is concerned largely with metabolism of the organisms, as in photosynthesis and pigmentation. Light is also a factor showing very frequent and marked fluctuations in the upper layers of the sea.
To animals, light has its greatest significance indirectly, as a source of energy for the photosynthetic processes of plants upon which all animals are wholly dependent for their nourishment. Aside from this, animal life may exist without solar light, as is witnessed by the presence of animals in the abyssal depths. Nevertheless, light or absence of light has been one of the most potent factors in the molding of structural development and in the adaptations of most marine animals. Light is also a great factor in the movement of animals.
Light and Color. The variegated colors, bandings, mottlings, and so forth, of many marine fishes living among the fronds and stipes of sea-weeds is a well-known phenomenon to anyone familiar with the sea. Likewise, various shades and patterns of gray and black are common, for instance, among tide-pool sculpins and the littoral flatfish of sandy or muddy bottoms. Many littoral crustaceans living upon seaweeds
In more recent publications Sumner (1934, 1939, 1940) has reviewed his own and other investigations pertaining to mechanisms of color changes and quantitative effects of visual stimuli on pigmentation, especially in fishes. The mechanisms that bring about these changes in color or shade are associated with the chromatophores or pigment cells, the contents of which are either activated directly by intensity of incident light or mediated through the eyes and nervous system in response to color or to albedo (proportion of incident light reflected) of the background. The pigments of the chromatophores produce degrees of shade or color by the dispersion or concentration of the pigment within the irregularly shaped chromatophore cell. A display of greater or smaller amounts of the pigment results, either evenly over the whole surface of the body or irregularly in patches, the resultant effect depending upon the degree of dispersion or concentration of pigment and upon the amount of pigment involved.
In the above we have referred mainly to a method of color change that is concerned only with rearrangement of the pigments already present. Such changes are usually quite rapid and may even occur within a few seconds, as is witnessed by reactions of the cephalopods in which color intensity may quickly shift from pale gray to a rich chocolate as the animal passes over a lighter or a darker substratum. Here light appears to be the stimulus activating the changes but, in addition, nervous reactions related to feeding or presence of enemies may also produce the temporary color changes in cephalopods.
A second type of color change involves gradual change in the quantity of pigment or in the number of chromatophores present. Experiments by Sumner and Doudoroff (Sumner, 1940) showed that during a period of about two months the amount of the pigment melanin produced in the guppy (Lebistes reticulatus) was nearly independent of the intensity of the light but was dependent upon the albedo of the background. The amount of melanin (or the number of melanophores) was found to be
Much might be written pertaining to protective coloration of animals, its physiology, evolution, and purpose, but ecologically the greatest significance that can be attached to the ability, possessed by some animals, to respond to their background by closely matching their own color with it, is the protection they must thereby gain in relation to their organic environment. It has been shown experimentally (Sumner, 1935) that certain fishes (Gambusia) adapted to blend with their background have a greater likelihood of survival from attack by predators than do fishes of the same type that are in contrast with the background. If we may trust our own visual sense as an index, we know that only the closest scrutiny can reveal the presence of some animals made practically invisible by the cloak of blending color or shade.
In what has been said, we have considered only littoral animals that live on or near a more or less illuminated background of solids, either the substratum or larger plants. There is, however, a great array of animals not normally provided with such a background. In the pelagic habitat, for example, the “background” is the surrounding water, and in the abyssal-benthic division the background is entirely devoid of solar light. In the pelagic division the aqueous surroundings have all degrees of light intensity, from sunlight at the surface to absolute darkness in the great depths. The quality of light is also different at different depths, since water does not absorb all light rays uniformly, but the Michael Sars Expedition found that in southern waters even the most penetrating rays failed to affect photographic plates exposed for two hours at a depth of 1700 m (p. 83).
It is of special biological interest to note that at a depth of 500 m the rays detected are not entirely diffused by the water but are directed so that shadows, however faint, should result. The dorsal side of an animal even at this depth is more exposed to light than the ventral or lateral sides and this may exert an influence, along with the force of gravity, in the orientation of the animals. To animals living at shallow depths the direction of light appears to be of great significance and most fishes of these zones are notably darker above than underneath. Investigators concerned with the effect of light on pigmentation have expressed the opinion that the shade assumed by an animal is chiefly determined by the ratio between the light from overhead and the light reflected by
Just how this principle of reaction to light would operate to affect the fishes and other animals in the open sea has not been shown, but in the zone where there is still considerable light there must be a difference in the light intensity from above and below. It appears that the above principle is best applied to conditions where the water is shallow and the background a well-defined reflecting surface with various values for the albedo. Be this as it may, there is still much evidence, especially from the findings of the Michael Sars Expedition in the North Atlantic, to show that there is a very real, though not satisfactorily explained, correlation between the light entering the sea and the color of the animals at various levels of light penetration.
The uniform dark pigmentation of fishes in the lightless depths, as contrasted with the ventral-dorsal differentiation of those inhabiting the lighted zone, indicates that the direction of light is significant in the distribution of pigment. But just why abyssal fishes do not become blanched in the absence of light as do cave fishes has not been satisfactorily explained.
It is generally known by mariners and fishermen that the animals living at or near the surface of the sea, especially in lower latitudes, are commonly blue in color. Such common fishes as the flying fish, mackerel, bonita, tuna, and others have backs of blue or blue-green. Some invertebrates, for example the siphonophore Velella and the pelagic molluscs Janthina exigua and Glacucus eucharis, are among the larger and better known, while copepod species of Pontellopsis, Anomalocera, and Corycaeus are frequent among the microscopic forms.
The blue and green colors are indeed striking characteristics of the surface open-sea life but it must not be concluded that all oceanic animals are blue or even predominantly so with respect to numbers. In many of the smaller forms, copepods and other crustaceans, pale red is a very common color, which like blue may occur as faintest tints in relatively hyaline forms. Hyaline or colorless animals (sometimes with the digestive tract or other parts colored) are as characteristic of the open sea as are the blue, or even more so, from near the surface to moderate depths (up to 200 to 300 m or deeper). Among these hyaline forms may be mentioned the salps Salpa and Doliolum; the arrow or glass worms Sagitta; the pelagic heteropod molluscs Pterotrachea and Atlanta; the Ctenophora or comb jellies; the copepods Eucalanus and Haloptilus; and many fish
larvae. The list could be extended almost indefinitely. The crystal clear aspect of many of these animals must make them practically indistinguishable to predators. Yet if this is a protective adaptation, we are left with the need of explaining why many species of these hyaline forms are so rare in comparison with others of the same type of animals that are more conspicuous owing to presence of color. As examples we may mention the hyaline versus the opaque or colored copepods. The latter are far more abundant as a rule. Perhaps the protective feature is coupled with a low rate of fecundity; or in plankton forms the unselective mode of feeding by predators may be yet another explanation since the hyalinity would be of little survival value.
At depths of about 300 to 500 m the silvery fishes, Argyropelecus (fig. 231-a), Chauliodus, and others, form a conspicuous though small part of the fauna, and there is a marked increase in reddish and dark-colored animals: dark fishes such as Cyclothone; many red crustaceans, wholly or partly colored, including, for example, the red prawn Acanthephyra, and numerous copepods such as Getanus, Metridia, Pleuromamma, and Euchaeta. The red Sagitta macrocephala and Eukronia fowleri of these depths are in contrast with colorless chaetognaths taken at higher levels. Other dark colors occur, including dark violet pteropods (Peraciles diversa) black or dark violet fishes (Gonostoma spp.), and brown medusae (Atolla).
In the deeper lightless waters, brilliant red, scarlet, or red-violet prawns (Acanthephyra spp.) persist, but the fishes are all black, black-violet, or brownish.
The depths at which these characteristically colored animals appear are not the same in all parts of the world. In waters of higher latitude the species of intermediate depths are nearer the surface than in tropical seas. Forms occurring at about 200 to 500 m in the higher latitudes, between about 67° and 50°N, will be found near 750 m at the latitude 33°N (Hjort, 1912). This difference indicates strongly a correlation with the degree of submarine illumination, for the upper limit of the dark pigmented forms agrees with the relative depths of light penetration at these latitudes. Further evidence is gleaned from the fact that in related species or varieties the darker and more heavily pigmented ones come from the greater depths. For example, among the fishes the light-colored Cyclothone signata has its lower limit near the upper limit of the black C. microdon. This is indicated by the following hauls taken at three depths by the Michael Sars at about 35°N.
Depth (m) | Light-colored C. signata, individuals | Dark-colored C. microdon, individuals |
---|---|---|
500 | 1240 | 214 (small) |
1000 | 82 | 448 |
1500 | 22 | 322 |
A similar vertical distribution was found for certain other forms of life. The deep-sea medusa Atolla bairdi has four varieties based on the degree of pigmentation. They range from a variety with pigment only in the stomach to the deepest dwelling variety with quite general pigmentation.
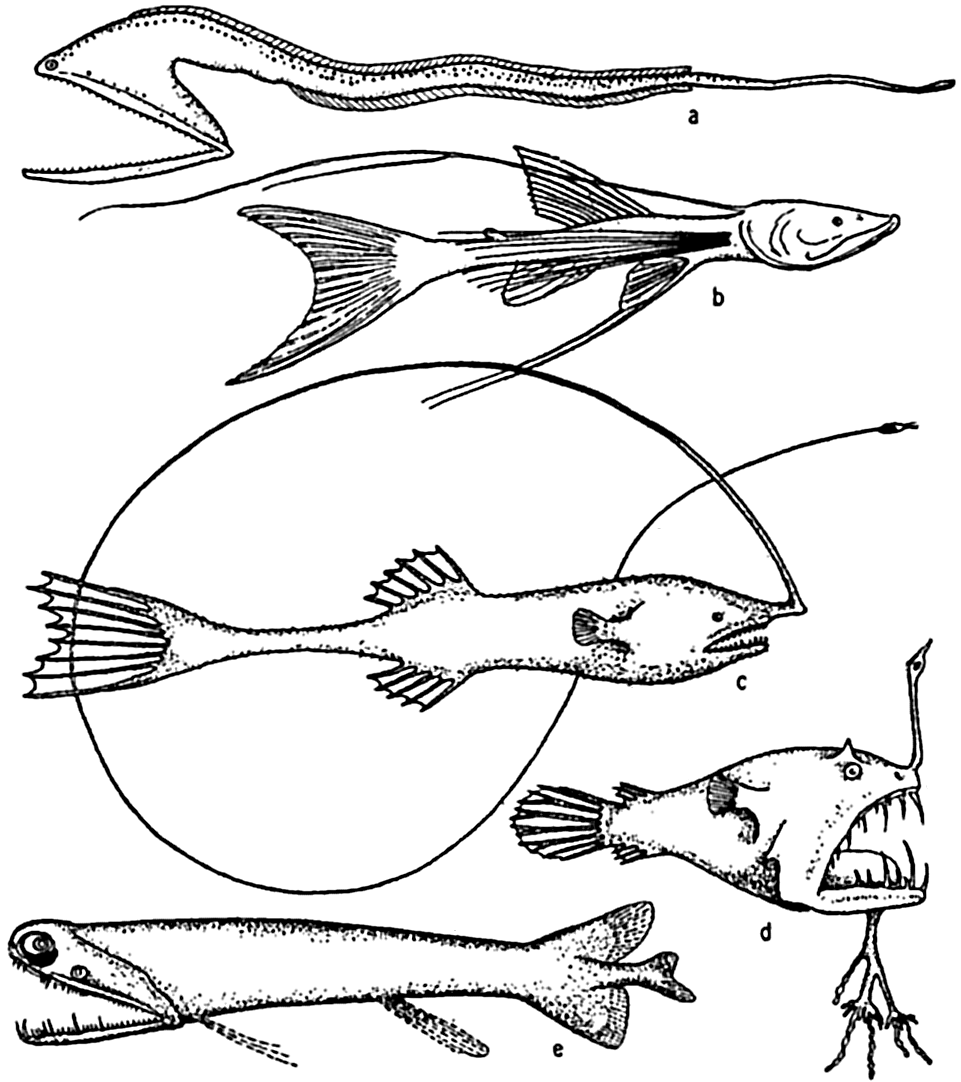
Deep-sea fishes: (a) Macropharynx longicaudatus (after Brauer), length 15.1 cm, from 3500 m depth; (b) Bathypterois longicauda (after Günther), length 7.6 cm, from 550 m depth; (c) Gigantactis macronema (after Regan), length 13.3 cm, from 2500 m depth; (d) Linophryne macrodon (after Regan), length 5.3 cm, from 1500 m depth; (e) Malacostus indicus (after Brauer), length, 8 cm, from 914–2500 m depth.
The red rays in the spectrum are quickly absorbed by the surface waters, hence, the animals below the surface live in a green, blue-green, or lightless world. In the abyssal regions it is indeed difficult to imagine what survival value any color can have, but near the surface, for example, the fishes with blue or green backs and silvery or light ventral sides are rendered thereby less conspicuous both from above and below, and the darker colors of fishes in dimly lighted depths also seem appropriate. In daylight red is a conspicuous color, but in the sea a red object appears quite black at a distance of only a few meters owing to the rapidity with which red rays are absorbed by water; and at depths below the penetration
Light and Structural Adaptations. That the quantity or quality of light has a marked influence on the coloration of marine animals inhabiting different depths of the sea seems beyond all reasonable doubt, but the influence of deep twilight or absence of light has indirectly placed a more remarkable stamp on structure than on color. This is manifest by the strange and weird anatomical adaptations, especially of many abyssal fishes.
These adaptations are concerned with structural modifications fitting the animals better to survive in faintest light or in utter and perpetual darkness. They are mainly along three lines: (1) tactile structures, (2) food-procuring contrivances, (3) light production.
Reference to publications dealing with abyssal fishes (Günther, 1887, Brauer, 1906, Murray and Hjort, 1912, Regan, 1932) will illustrate how characteristic are modifications along these lines. Development of tactile devices is an important feature in numerous instances and is well illustrated in Macropharynx longicaudatus, several species of Bathypterois, Gigantactis macronema, Linophryne macrodon, and other deep-sea angler fishes (fig. 230). These sensory structures may, as indicated in the figures, involve modification of the whole body whereby it is elongated and ends in a disproportionately long threadlike tail as in Macropharynx; or, in the more conventional bodies, the fin-rays may grow to a length several times that of the body; or tactile threads of various types may grow directly from the head. The prawns of deep water also possess extremely long tactile antennae, some of which may be twelve times the length of the body as in Aristaeus aristaeopsis.
The food-procuring contrivances of deep-sea fishes consist commonly of an extraordinarily immense mouth in proportion to the body size. The stomach and abdominal wall may be so elastic that it is possible for some of these fishes, Cheasmodus or Melanocetus for example, to swallow other fishes three times their own size and many times their own weight. Many of the deep-sea fishes are provided with formidable teeth, attesting to their carnivorous nature. As if this were not enough, some species of deep-sea angler fishes possess a tactile organ, the illicum, useful as rod and line, and provided with a terminal luminous lure, which in some cases may even be armed with hooks, as is the case with Lasiognathus (Regan, 1926; fig. 231-b). These angling processes may be four times as long as the fish, as in Gigantactics macronema (fig. 230-c). In food habit these fish must be mainly piscivorous. The possession of these unusual devices is correlated with the scarcity of food in the deep-sea pelagic
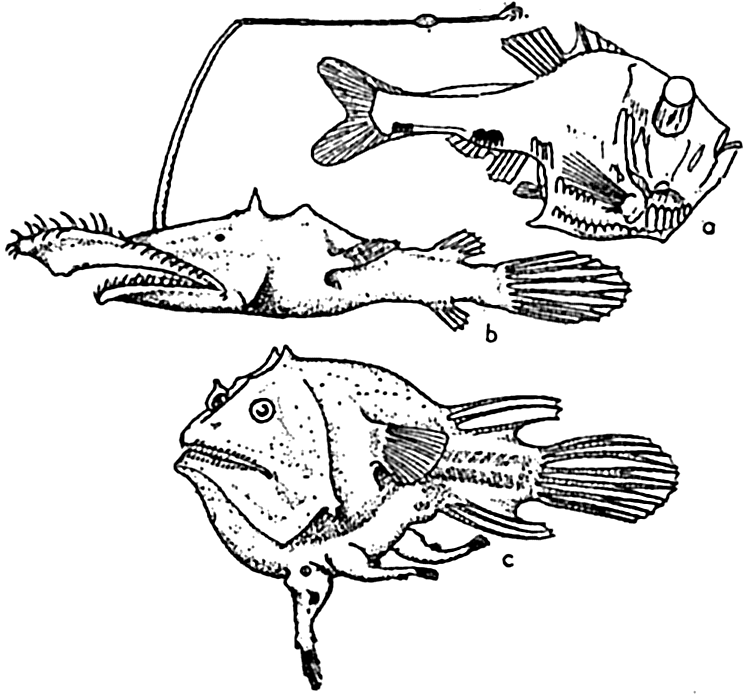
Deep-sea fishes: (a) Argyropelecus hemigymnus (after Hjort), length 3.5 cm, from 300 m depth; (b) Lasiognathus saccostoma (after Regan), length 7.5 cm, from 2000 m depth; (c) Edriolychnus schmidti (after Regan), length 7 cm, from 2000 m depth.
We have but little information regarding the depth at which fishes and other animals can see, but for purposes of comparison the question may be raised as to the depth at which light is still perceptible to the human eye. The human eye appears to respond to an intensity of light about 10−10 times that of bright sunlight, the greatest sensitivity at low intensities lying in the green at wave length .503 μ. One can easily find the depth at which light is still perceptible by making use of the absorption coefficients at .503 μ which have been found for the different types of water (p. 84). These depths, based on Utterback's data (1936), are entered to the left in table 97, and to the right in the same table are entered corresponding values which Clarke has computed from his observations. In the clearest oceanic water light should still be perceptible at a depth of 700 m, whereas in average oceanic water the corresponding depth would be 300 m, and in turbid coastal water 60 m. The values agree well with those of Clarke (1936), who finds that in the Sargasso Sea light is perceptible down to 430 m, whereas in Woods Hole Harbor light could not be perceived below a depth of 75 m if the waters were that deep. Clarke compares his results with Beebe's observations from the bathysphere, according to which complete darkness for the human eye was reached at a depth of between 520 and 580 m. Helland-Hansen's observations indicate that even at these depths light is directed, wherefore weak shadows will be present. According to the experiments by Grundfest (1932), the sunfish Lepomis can see at illumination as low as that
Type of water (from Utterback's data) | Depth (m) | Region (from Clarke) | Depth (m) |
---|---|---|---|
Pure sea water. | 1530 | ||
Clearest | 700 | Sargasso Sea | 430 |
Oceanic water Average | 300 | Deep basin, Gulf of Maine | 230 |
Turbid | 158 | Georges Bank | 180 |
Clearest | 115 | Off Gray Head | 130 |
Coastal water Average | 80 | Woods Hole Harbor | 75 |
Turbid | 57 |
In a world of much reduced light or absolute darkness, it is to be expected that the organs of vision will be among those modified, either towards ends resulting in better utilization of the faint rays of solar or biological light that may be present, or in diminution or entire loss through disuse. Both of these modifications have occurred, even in forms that are closely related. The former has been accomplished in some instances by enlargement of the eyes to receive better the faint rays of light, and by increase of visual cones and loss of the rods in the retina. The cones are the functional cells for dim light. Reduced light appears also to have led in some instances, in both fish and cuttlefishes, to the development of “telescopic” eyes, that is, large-lensed eyes that protrude from the sockets as in Argyropelecus (fig. 231-a), indicating binocular vision. This type of adaptation is not an isolated one but is said to occur in five orders and several suborders of fishes.
The conditions of light in the deep sea have not invariably led to a convergent evolution providing more efficient eyes according to the above modifications; many animals, especially the bathypelagic fishes (but also crustaceans, at least one species, and a squid), have evolved species that are blind or have degenerate eyes, apparently atrophied from disuse.
Bioluminescence. In the biological world, heat and motion are common expressions of energy but electricity and light are also produced, especially by marine animals. Bioluminescence, popularly spoken of as “phosphorescence” because of an earlier belief that it was caused by the element phosphorus, is primarily a phenomenon of the sea. It is not exclusively confined to the sea, to be sure, but is overwhelmingly more abundant there than anywhere else. No light is known to be produced by fresh-water animals with the exception of one aquatic glowworm, and in terrestrial organisms the production of light is relatively rare. In the sea, the power to emit light is not confined to any particular taxonomic group of animals but is so generally distributed that most major animal groups are involved, from the protozoans to the vertebrates. Many marine bacteria also are luminescent.
The number of animal species capable of emitting light runs into many thousands, distributed among such groups as the radiolarians, dinoflagellates, hydroids, jellyfishes, alcyonarians, ctenophores, bryozoans, polychaete worms, brittle stars, many crustaceans (including ostracods, copepods, schizopods, and decapods), gastropods, bivalves, cephalopods, protochordates, and true fishes. Harvey (1920, 1940) should be consulted for a fuller discussion of these. It is highly probable that many groups not now known to be luminescent will prove to be so as our information becomes more complete. Only recently the nemerteans have been reported to have members that luminesce. Light producers are found in all marine communities from the surface of the sea to abyssal depths. As far as surface display is concerned, luminescence is more common in tropical than in northern latitudes, although it may be very striking in boreal waters during the warmer seasons. But this is associated in part with the fact that many luminescent organisms of the surface, especially the dinoflagellates, are mainly thermophiles, requiring
The light rays produced by organisms are wholly within the range of human vision and may at times be sufficiently brilliant to make the crests of breaking waves, the wake of a ship, or other mechanically agitated water glow with a general greenish light of sufficient intensity to enable one to read. This general phenomenon wherein the water itself appears to glow is caused by innumerable microscopic organisms, mainly dinoflagellates of various types, such as Ceratium or Noctiluca (fig. 225-g). Scattered throughout the glowing water are the larger, brighter points of flashing light emitted by jellyfish, copepods, euphausiids, annelids, or other larger forms.
In many of the luminescent organisms the light results from luminous slime secreted over parts of the body or thrown out as a glowing cloud about the animal, as in the case of the squid Heteroteuthis, which produces this secretion in a gland corresponding to the ink sac of better known squids. Many of the fishes and crustaceans possess very highly specialized luminous organs which in some cases are under nervous control. These organs are provided with light-producing cells, reflector, pigment layer, and lens. It has been observed (Macdonald, 1927) that six specimens of the crustacean Meganyctiphanes norvegica in a two-liter jar voluntarily emitted sufficient light for reading news print.
Only in relatively few instances can we perceive a usefulness of the light to the organism producing it. In dinoflagellates, bacteria, jellyfish, hydroids, and so forth, there appears to be no possible utility; but in the higher forms, particularly those with specialized light organs capable of flashing under nervous control and arranged in definite patterns and even specific colors, utility seems clear. Light can have meaning in these instances only if eyes are developed to see it. In this respect it is significant to note, as has been pointed out by Hjort (1912), that pelagic fishes living above 500 m have both well-developed eyes and luminous organs, while in the bathypelagic forms below 500 m both types of organs are diminished. An apparent anomaly then occurs at the bottom, where the eyes of the fishes are well developed but luminous organs are scarce
Confronted with the information which we now have on the distribution of organisms with eyes and on the highly complicated structures for light production (Brauer, 1906), we cannot but believe that luminosity is of specific use to many animals, although in many forms such as bacteria and protozoans its inception may have been only a secondary physiological manifestation.
The biochemical activities involved in bioluminescence are only in part understood. It is a highly efficient process since the light is practically without heat and is entirely within the spectral region of human vision, there being no rays of infrared or ultraviolet. The light is produced by oxidation of a substance, probably a simple protein known as luciferin, that is produced by the living cells but that may function even though separated from the cells. However, before light can be produced by union with oxygen it is necessary that another substance, luciferase, be present as a catalyst to accelerate the oxidation.
It has been suggested (Pierantoni, 1918) that wherever light is produced the actual agents are bacteria living symbiotically with the animals, but there is evidence that this is not universally true (Harvey, 1920).
Bioluminescence has been discussed at some length because, in commanding the attention of ecologists, morphologists, physiologists, bacteriologists, chemists, and physicists alike, it is representative of the problems of marine biology.
Vertical Migrations. Light is a significant factor in the behavior of animals both pelagic and littoral. It is therefore an especially important factor in the local or restricted (as opposed to geographic) distribution of animals. In the intertidal zone most animals are found in shaded situations, in crevices, or on the under sides of rocks or ledges. Much caution must be exercised, however, in attributing this type of distribution
The correlation of animal movements with light is best studied in the pelagic division of the ocean and we shall limit our discussion mainly to the animals of this environment. Numerous investigations of the vertical distribution of plankton organisms of the open sea have shown that many, if not most, of the pelagic forms make daily journeys from deeper water to the surface at the approach of darkness and begin returning to deeper water at or before the break of dawn. This rhythmic movement is known as diurnal migration. See Kikuchi (1930) for a review of various factors that have been considered as motivating forces. The range of depth covered and the time of movement vary with different species of animals. Those forms capable of moving vertically through several hundred meters of depth, whether in diurnal or irregular movements, are the eurybathic animals with a wide range of tolerance to rather rapid changes in pressure. Since the range of temperature may be considerable they may also be eurythermic.
A great amount of investigation has been carried on with reference particularly to diurnal migrations of pelagic copepods and chaetognaths, and these may be considered as typical of much of the plankton community and its movements.
The population does not accumulate and move in a compact body; rather, it shows a more or less continuous but uneven vertical distribution that sometimes extends over two or three hundred meters in depth. The greatest number of individuals will be found concentrated at a level where conditions are optimum with respect to light in relation to other factors. That portion of the population found at given distances below or above the optimum may consist of individuals of uniform age or sex (Russell, 1927, Gardiner, 1933, Nicholls, 1933). In one investigation (Nicholls, 1933) of a mixed population of Calanus finmarchicus, the adult females exhibited diurnal migrations to a greater degree than other members of the population. The following data will serve to illustrate the general nature of migrations in that species (fig. 232):
It will be seen that at 4:00 p.m. on January 24th the females were massed between 30 and 80 meters. The sun set at 4:27 p.m. and by 7:00 p.m. most of these females had moved into the upper 30 meters of water. Throughout
― 837 ―the investigation a small proportion remained in the deeper water irrespective of the migration of the bulk. By 10:00 p.m. the accumulation in the upper layers was more marked, but by 1:00 a.m. the descent had begun and females were evenly distributed from the surface down to 80 meters.At 4:00 a.m. they were concentrated between 30 and 60 meters with none at the surface. By 7:00 a.m. they were found between 30 and 80 meters. The sun rose at 8:37 a.m. and by 10:00 a.m. the upper layers were quite deserted; most were found between 60 and 80 meters, but a large proportion was distributed below 80 meters, and 4:00 p.m. on the 26th saw a return to the condition in which they were found at that time on 25th.
The more even distribution at 1:00 a.m. is interpreted as indicating the beginning of descent following complete removal of the stimulus of diffused light, but this scattering or descent apparently does not always occur until return of light at dawn (cf. Metridia, fig. 233; Clarke, 1933).
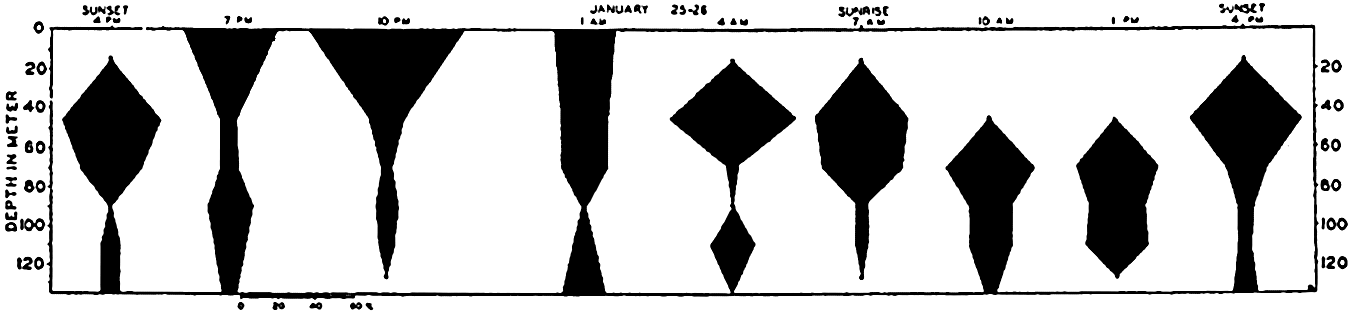
Diurnal migrations of adult female, Calanus finmarchicus (from Nicholls).
Not only do different species react differently, but different stages of development, and the sexes also, have their own characteristic behavior with respect to diurnal response associated with light. A further complication is encountered in that the degree of response is also seasonal, indicating changes in physiological state (Russell, 1928). Species of Acartia give some indication of a diurnal physiological state which causes migrations even in the absence of light (Esterly, 1917).
Since an animal is constantly subjected to various and simultaneous stimuli, some of fluctuating intensity, the nature of the movement will depend upon the combination at any one time, and it is not always clear which is the dominating stimulus.
In attempts to analyze these daily migratory habits several views have been expressed. It is agreed that light is the chief motivating factor, but we do not know whether it operates by virtue of its absolute magnitude of intensity or by change in intensity (Clarke, 1933, Johnson, 1938). Also it is not yet clear whether light evokes response directly or only indirectly through its modification of response to such factors as gravity, temperature, and food. The chief directional stimuli that plankton animals can receive are gravity and light, but the response to these may be modified by temperature, salinity, hunger for food, and so forth.
Gravity is an invariable factor constantly in operation, a force of momentous importance in the life of planktonic organisms. If it is not favorably overcome within water layers offering tolerable living conditions for the organisms it must result in their ultimate destruction in lower water layers or on the bottom. Some of the means employed to minimize this rate of sinking by wholly or relatively passive forms have been discussed elsewhere (p. 821). It must be emphasized that swimming upwards (negative geotropism) is of extreme importance, even to such weakly motile animals as copepods. But as observations have shown that plankton organisms have a twenty-four-hour cycle in which upward and downward migrations occur, it has been suggested that the intensity of light may be the agency affecting a change in the sign of geotropism (Esterly, 1917, 1919, Clarke, 1934). Thus in bright light the reaction to gravity is positive and the animals not only sink but may actually swim downward. In the comparative darkness of greater depths the effect of light becomes progressively less compelling and a threshold is finally reached, as is shown by a maximum density of animals at some depth. At midday this maximum will be found at its greatest depth. A reduction in light intensity with the approach of evening reverses the geotropism and the population spreads upward even to the surface. However, on approaching the surface, the population may sometimes encounter a sharp gradient of temperature, the thermocline, that may supply a sufficiently strong stimulus to dominate the field of stimuli and prevent further ascent toward the surface. Likewise a sharp thermocline limits the downward distribution of some surface forms. Observations in the Gulf of Maine show that the maximum number of Metridia lucens generally ascends to the thermocline, which has a gradient of about 7°C in 10 m, but does not pass through it (fig. 233); and Centropages typicus, which inhabits the warm-water stratum from 10 to 20 m, does not in general go below the thermocline into water of 8° to 5°C (Clarke, 1933).

Diurnal migrations of the adult female copepod, Metridia lucens. The changes in light intensity are indicated by lines representing the depth at which 1000 microwatts, 100 microwatts, and so forth, occurred (from Clarke).
Since most of the microplankton and diatoms upon which larger plankton forms subsist are produced in the euphotic layer, it has also been suggested that upward migrations may be made in search of food when light intensities are not so great as to be prohibitive (Worthington, 1931).
The phenomenon of diurnal migrations is not confined to animals living relatively near the surface. Sergastid prawns, for example, have their maximum abundance between 0 and 200 m at night and between 600 and 800 m during the day (Welsh, Chace, and Nunnemacher, 1937). We learned earlier that light is directional even at these depths and could conceivably be the directing force in very light-sensitive forms, as these must be. Dark-colored deep-water fishes were taken by the Michael Sars at the surface during the dark hours, whereas during the day they occurred only in the depths of much reduced light. The whole problem of vertical migrations is complex; and although we know that extensive vertical migrations occur with recurrent external conditions, yet they are variable in extent with respect to place and season, and need much additional investigation.
Other vertical migrations are discernible as general seasonal movements of certain populations. These movements are associated with critical periods, breeding for example, in the life cycle of the species involved rather than with light, and are best discussed elsewhere (p. 322). Since reactions related to spawning may be more or less spasmodic and contrary to the regularly observed reactions, so that extensive swarms may be found at the surface even during the daytime, they must be kept in mind in the interpretation of vertical movements.