Hydrothermal Alteration
Hydrothermal alteration is a general term embracing the mineralogical, textural, and chemical response of rocks to a changing thermal and chemical environment in the presence of hot water, steam, or gas (Henley and Ellis, 1983). By mapping alteration mineral assemblages at the surface (but more commonly within drillholes), it is possible to locate the zones with highest temperatures, pressures, or permeabilities—all of which are important in geothermal exploration. The same techniques are used to map fossil hydrothermal systems associated with epithermal ore bodies. Epithermal is a
|
mining term that refers to a hydrothermal mineral deposit (fossil hydrothermal system) formed within 1 km of the Earth's surface in the temperature range of 50 to 200°C (Park and MacDiarmid, 1970).
Figure 3.8 shows the cycle of solution for rocks during water/rock interaction and the deposition of hydrothermal minerals in pore space, both of which greatly affect the physical properties of reservoir rocks and make up the hydrothermal cycle (Elders, 1981). Leaching and fracturing reduce the bulk density and increase porosity and permeability, whereas the deposition of hydrothermal minerals increases the bulk density and decreases porosity.
Characterization and Interpretation
The two basic types of alteration associated with volcanic geothermal systems, acid-sulfate and adularia-sericite are modeled in Fig. 3.9. Acid-sulfate alteration occurs within the uppermost parts of a volcano or along caldera ring fractures where there is abundant, cool groundwater; acid-sulfate water is formed where the groundwater mixes with rising magmatic gases. Adularia-sericite alteration occurs within a flow regime high above or adjacent to a deep heat source and is characterized by neutral pH and alkalichloride waters (Heald et al ., 1987). Alteration rank , used as an empirical indication of temperature and permeability within a volcanic field, is determined through studies of secondary minerals; for example, epidote is an indicator of high temperature and adularia is characteristic of high temperature and high permeability within a hydrothermal system (Browne, 1977).
Many terms used to describe alteration assemblages have evolved in the literature of both geothermal and ore-deposit exploration. Heald et al . (1987) evaluated and correlated these terms and their uses in the two fields to generate the material presented in Table 3.2.
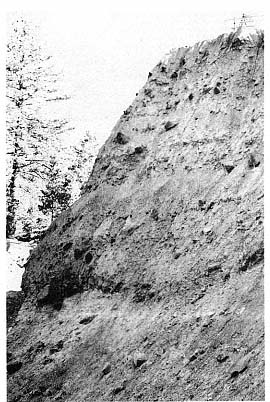
Fig. 3.7
Phreatic explosion breccia on the east wall of
South Crater, Inyo Craters, California. South
Crater is a 100-m-diameter phreatic (and
possibly partly phreatomagmatic) crater that
overlies both rhyolitic and basaltic dikes.
Deposits here are 25 m thick and consist of a
poorly bedded, cross-bedded lithic ash that
contains blocks up to 0.5 m in diameter (lower
half of the deposit) and massive, block-
bearing coarse ash (upper half of the deposit).
Browne (1977) described 51 hydrothermal minerals found in active geothermal systems; some of these minerals also occur in low-grade metamorphic rocks. The water/rock interactions in the system result in alteration of, first, volcanic glass and then a sequence of mineral phases—replacing them, leaching them, or depositing new minerals in available pore space (Browne, 1982). Typical alteration replacement products are listed in Table 3.3. The mineral assemblage depends on temperature, pressure, fluid composition, and permeability,
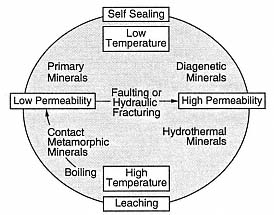
Fig. 3.8
The hydrothermal cycle. This diagram
demonstrates the interplay of water/rock
interaction, deposition of hydrothermal
minerals, and fracturing in a constantly
evolving hydrothermal reservoir.
(Adapted from Elders, 1981.)
and the sequence of mineral alteration and replacement varies from system to system, as is shown in Table 3.4 for several hydrothermal systems.
There is a general relationship between temperature and mineralogy for aluminosilicate alteration minerals (see Fig. 2.47), and mineral suites can be used to interpret temperatures within a geothermal system (Fig. 3.10; Henley and Ellis, 1983). For example, the minerals epidote and wairakite do not appear until 200°C.
During his examination of the geothermal field at Broadlands, New Zealand, Browne (1970) determined that many hydrothermal minerals are of little use in estimating subsurface temperatures and permeability; among these are chlorite, pyrite, calcite, and quartz, which are stable over a wide temperature range. Calcite is strongly affected by underground CO2 pressure. Mordenite, siderite, and cristobalite, which form at low temperatures, and epidote, which forms at high temperatures, are not greatly affected by permeability. Clays, which are excellent indicators of temperature, are not good
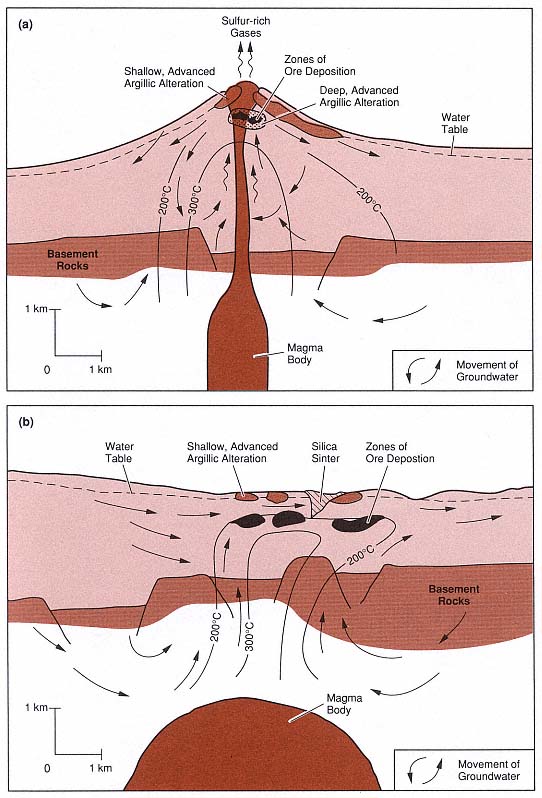
Fig. 3.9
Models of two types of fossil hydrothermal systems that are responsible for epithermal ore deposits.
(a) In the system characterized by acid-sulfate alteration, wiggly arrows represent rising sulfur-rich
magmatic gases; these gases condense and oxidize to form the acid fluids responsible for leaching
and argillic alteration of rocks within the volcano and at the surface. (b) In the system characterized
by adularia-sericite alteration, alkali-chloride waters have a neutral pH.
(Adapted from Henley and Ellis, 1983, and Heald et al ., 1987.)
|
|
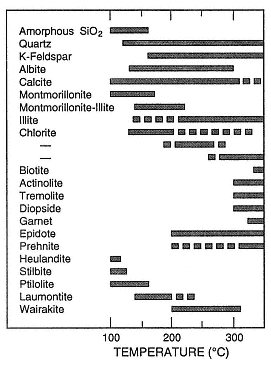
Fig. 3.10
Summary of temperature ranges for common
aluminosilicate minerals. Solid lines indicate the
most common temperature ranges for these
occurrences. The three ranges shown for chlorite
are related to the transition, with rising
temperature, from swelling chlorite through
mixed swelling and nonswelling
chlorite to nonswelling chlorite.
(Adapted from Henley and Ellis, 1983.)
|
guides to permeability. Browne (1970) found that the most important minerals in hydrothermal surveys are the feldspars, which are sensitive to temperature and permeability. Andesine (the most common feldspar at Broadlands) is altered at temperatures between 70 and 290°C, depending on permeability, to quartz, clay, calcite, albite, or adularia. Albite replaces andesine above 230°C. Adularia may replace andesine completely or may be mixed with albite. Good production zones contain abundant secondary quartz, adularia, and calcite (Table 3.5). At Broadlands, there is a correlation between high steam and water production and the presence of adularia as the dominant feldspar in the reservoir rocks. Browne and Ellis (1970) noted that pyrrhotite occurs above 180°C but is limited to impermeable zones.
In near-surface steam-heated zones of acidsulfate geothermal systems, underground boiling adds dissolved magmatic gases to the steam phase, and oxidation creates an acid condensate above the boiling zone (Henley and Ellis, 1983). The mineral assemblages that are characteristic of acid alteration include kaolinite, alunite, gypsum, opal, and hydrated iron oxides (Steiner, 1977); this assemblage is referred to as advanced argillic alteration.
Temperatures decrease and pH increases outward from the central portion of an acid hydrothermal system, producing a systematic variation in stable mineral assemblages that can be mapped horizontally—or vertically if there are coreholes. These trends are shown in Table 3.6 (Hayashi (1973) and in Fig. 3.11 (Heald et al ., 1987).
Mapping Alteration Mineralogy
The Geological Survey of Japan considers mapping hydrothermal alteration zones an extremely important element in geothermal exploration. Although springs and fumaroles are the most obvious surface manifestations of the hydrothermal system,
|
alteration zones supply additional information that points out the areas of greatest temperature and permeability. Alteration zones can also guide exploration geologists to hidden systems or to ancient spring activity. The mapping process involves systematic sampling across the study area and analysis of mineral phases by x-ray diffraction and petrography. Study areas can range from a general map of altered areas over hundreds of square kilometers—which will often show the relationship of hydrothermal systems to large features such as calderas—to small areas of less than 1 km—where detailed variations in alteration can be documented.
An example of this type of exploration technique is the eastern Hachimantai Geothermal Area, Honshu, where there are many geothermal areas scattered throughout an 800-km2 volcanic field (Geological Survey of Japan, 1986; Nakamura et al ., 1981). Within the field, Nakamura et al ., have established three alteration subzones.
· Silicic subzones are characterized by porous, white or brown siliceous rocks that contain small amounts of alunite and sulphur. These subzones are usually found in the center of the system and can be evidence of strong hydrothermal activity in the past.
· Silicification subzones consist of hard, white, silicified rocks within a band around the silicic zone. This subzone can occur as blocks 0.5 by 0.7 km or as 10- to 50-m-wide veins. The minerals include saponite, chlorite, hydromica, mixed-layer clays, sericite-montmorillonite, alunite, anhydrite, gypsum, calcite, rutile, diaspore, and andalusite.
|
· Argillization subzones are the outer-most zones of alteration; they consist of blue-black clays (the color is mostly related to finely disseminated pyrite). The dominant minerals are montmorillonite, kaolin, and alunite, in order of distance from the outer edge of the zone.
In addition to these alteration zones, a pyrophyllite zone, which may overlap the argillized rocks, has formed at higher temperatures and may be an indicator of higher permeabilities. Pyrophyllite is most likely formed within the system if temperatures are >300°C and if the geothermal fluids are acidic at depths of ~1 km.
Figures 3.12a and 3.12b show the distribution of alteration zones, which are identified by the dominant mineral phase, as well as the distribution of fluorine concentrations (another exploration tool). The schematic cross-section of B—B' in Fig. 3.12c was based on wells that were drilled into the vapor-dominated part of the Matsukawa geothermal field; this illustration shows a relationship between the reservoir and surface pyrophyllitic to kaolinitic alteration zones. Wells for the Kakkonda (Takinoue) geothermal field penetrated a water-dominated reservoir in a zone where rocks are mostly altered to montmorillonite (on a regional scale) but are locally altered to kaolinite, alunite, or pyrophyllite.
The examples from Nakamura et al . (1981), cited earlier, are sited in intermediate to silicic calc-alkaline rocks. However, different

Fig. 3.11
Diagram showing the alteration minerals in vein assemblages and the sequence of wall-rock
alteration for acid-sulfate and adularia-sericite-type deposits that occur in fossil hydrothermal
systems. No scale is given because the widths of alteration zones range from
centimeters to tens of meters outward from the vein.
(Adapted from Heald et al ., 1987.)
mineral zonation occurs in basaltic rocks. For instance, Tómasson and Kristmannsdóttir (1972) described three vertical zones in the Reykjanes geothermal area of Iceland, which are listed here in order of increasing temperature:
(1) a montmorillonite-zeolite-calcite zone,
(2) a mixed-layer clays-prehnite zone, and
(3) a chlorite-epidote zone.
The zones are not always clearly defined as a result of cooling and reheating after the invasion of sea water. Subsurface temperatures at a depth of 1 km exceed 200°C.
The date and length of hydrothermal activity in a geothermal system can be determined through potassium-argon dates for clays (Woldegabriel and Goff, 1989). These data can provide interesting and sometimes crucial information on the longevity (and perhaps future) of hydrothermal activity in an area to be drilled and developed. Woldegabriel and Goff (1989) have shown that hydrothermal systems within the Valles caldera of New Mexico became active soon after caldera collapse at 1 Ma and have been active from that time to the present.
The most desirable targets—permeable zones with hot water and steam—are narrow ones and, in some cases, may make up as little as 5% of the entire geothermal system. The size of this target can vary substantially. Figure 3.13 compares 16 epithermal ore bodies (fossil hydrothermal systems; Heald et al ., 1987) and 25 geothermal fields (Rowley, 1982). The areas of geothermal fields, from 0.15 to >100 km2 , are very similar to the areas of epithermal deposits, which range from 1 to >120 km2 . The projected surface areas of production zones within the 16 geothermal fields range from <0.5 to 60 km2 , which is 5 to 15% of the total area defined by hydrothermal activity, rock alteration, and elevated geothermal gradients. These areas
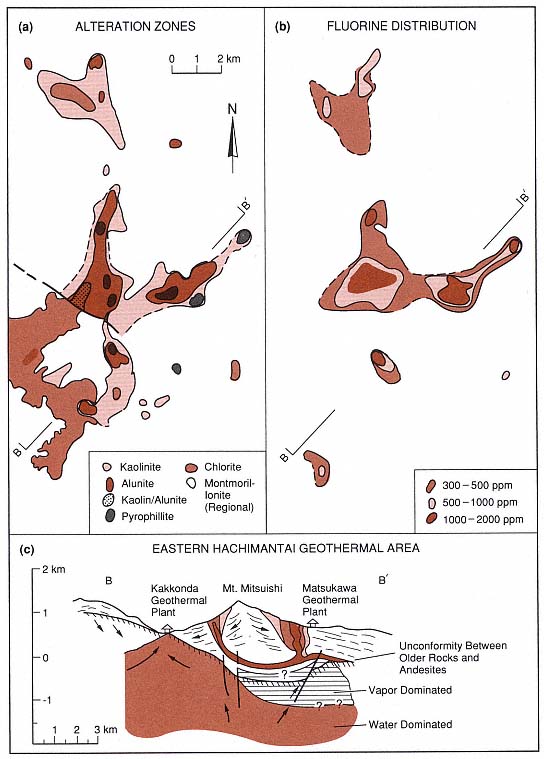
Fig. 3.12
Maps of alteration zones from the eastern Hachimantai geothermal area in Japan. (a) Map of
alteration zones indicates the predominant marker minerals. (b) Distribution of fluorine in
hydrothermally altered rocks. (c) Schematic cross-section of
the eastern Hachimantai geothermal area.
(Adapted from Geological Survey of Japan, 1986.)
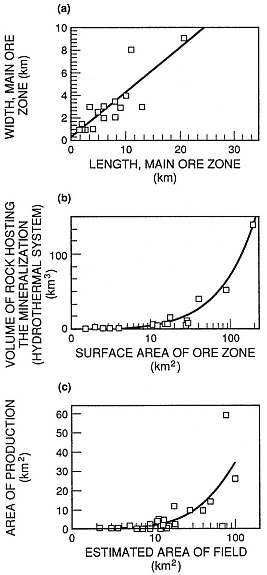
Fig. 3.13
Dimensions and volumes of hydrothermal systems
are used to compare ore zones (fossil hydrothermal
systems) and developed hydrothermal reservoirs.
These graphs provide a general idea of the range
of areas and volumes for hydrothermal reservoirs.
(a) Length and width of ore zones. (b) Surface areas
of ore zones and volumes of fossil hydrothermal
systems. (c) Estimated areas of geothermal fields
and actual areas with production wells.
(Adapted from Heald et al ., 1987,
and Rowley, 1982.)
of production are the permeable pathways for hot fluids at the time of drilling. By analogy, epithermal ore deposits encompass the entire area affected by alteration throughout the history of the hydrothermal system; large ore-bearing veins were the main conduits for geothermal fluids and gases. By studying the analogy between active hydrothermal systems and epithermal ore bodies, it is possible to create three-dimensional models of volcanic geothermal systems. The depth of hydrothermal reservoirs ranges from <1 km to perhaps as much as 4 or 5 km. The depths of some of these reservoirs have not been determined. Mining epithermal ore deposits has provided us with the vertical extent and time-cumulative volume of many such ore deposits; they are from 400 to 1,000 m vertically and have volumes of 1 to 132 km3 (Heald et al ., 1987). The largest geothermal systems and epithermal ore bodies are associated with the Earth's largest volcanoes—calderas—which are discussed in the next chapter.