4.4—
Photosynthesis
In the process of photosynthesis in green plants, light energy is absorbed by the pigments of the chloroplast and converted into chemical free energy in the form of ATP and NADPH, which are then used for the reduction of carbon dioxide and the synthesis of plant materials. The major organic products of photosynthesis are carbohydrate and the overall equation of photosynthesis is:

The oxygen which is evolved is derived from water. Carbon dioxide is converted to carbohydrate via the Calvin cycle of reactions (Calvin & Bassham, 1962). In the carbon reduction cycle, one molecule of ribulose bisphosphate (RBP or RuDP) reacts with CO2 to form two molecules of 3-phosphoglyceric acid (PGA), which are converted into phosphoglyceraldehyde (triose phosphate) in a reaction which needs two molecules of NADPH and two molecules of ATP. The regeneration of the CO2 acceptor (RBP) involves a complex series of reactions and requires one molecule of ATP. The overall requirement of the Calvin cycle is 3 molecules of ATP and 2 molecules of NADPH for each molecule of CO2 reduced to carbohydrate. In the C4 -dicarboxylic acid pathway of photosynthesis, in which CO2 reacts initially with phosphopyruvate, 5 molecules of ATP and 2 molecules of NADPH are needed for each molecule of CO2 reduced (Hatch & Slack, 1970).
The light reactions of photosynthesis and the formation of NADPH and ATP are performed by the thylakoids, and the carbon reduction cycle occurs in the stroma or soluble phase of the chloroplast.
4.4.1—
The Photosynthetic Unit and Energy Transfer
The light absorbing molecules, i.e. the chlorophylls and carotenoids, are organized into units in the thylakoid membrane. Quanta absorbed by a large number of pigment molecules are transferred by an efficient resonance mechanism to a special molecule of chlorophyll a , called the trap or reaction centre chlorophyll, where the primary conversion of light energy into chemical free energy takes place. This is the concept of the photosynthetic unit which was first proposed to account for the observation that the maximum yield of CO2 fixed, or O2 evolved, per single flash of intense light was one mole per 2,500 moles of chlorophyll. The absorption band of the reaction centre chlorophyll a is at a longer wavelength than the absorption bands of the light-harvesting pigments to ensure efficient trapping of the energy at the reaction centre.
The reaction centre chlorophyll is in close association with an electron acceptor molecule (A) and an electron donor molecule (D) in the thylakoid membrane. On excitation of the reaction centre chlorophyll (Chl*) by transfer of energy from the 'antenna' pigments, an electron is donated to A giving A– and leaving the chlorophyll molecule deficient in an electron (eq. 4.1). The positively charged chlorophyll then receives an electron from the donor D, and is restored to its ground state energy level (eq. 4.2). The net result is that the energy of a photon is used to transfer an electron from D to A, and thus the primary photochemical event of photosynthesis is an oxidation-reduction process.

4.4.2—
Two Photosystems and the Z-Scheme of Electron Transport
Investigations in the 1950's and early 1960's established that the thylakoid membrane contains two types of photosynthetic units, designated photosystem 1 (PS–1)and photosystem 2 (PS–2), which cooperate in a sequential manner to transfer electrons from water to NADP+ (Boardman, 1968). Figure 4.4 depicts the electron transport pathway known as the Z-scheme first put forward by Hill and Bendall (1960). Both photosystems contain chlorophyll a , chlorophyll b and the four carotenoids, but in different proportions. Each unit of PS–1 or PS–2 contains 200 light-harvesting chlorophylls and one reaction centre.
Quanta of light absorbed by PS–2 are transferred to a form of chlorophyll absorbing at 682 nm and termed chl a– 682. Excitation of chl a– 682 catalyses
the transfer of an electron from Y to Q, giving a strong oxidant, Y+ , and a weak reductant, Q– . Oxidation of water and the release of a molecule of O2 requires the sequential absorption of four quanta and the accumulation of four oxidizing equivalents (Cheniae, 1970). The mechanism of water oxidation is unknown, although it is established that manganese, probably in the form of a manganeseprotein complex, and Cl– are required (Boardman, 1975). The primary acceptor of PS–2, (Q), has a redox potential around zero volts, and is incapable of reducing NADP+ , without an imput of energy into PS–1. Quanta absorbed by PS–1 are transferred to P–700, a form of chlorophyll a absorbing at 700 nm. On excitation, P–700 donates an electron to an acceptor Z, resulting in the formation of P–700+ , a weak oxidant with a mid-point potential of + 0.43V, and Z– , a strong reductant with a redox potential in the vicinity of –0.6V. P–700+ interacts with the weak reductant, Q– , generated by PS–2 via an electron transport chain, which includes plastoquinone A, cytochrome ¦ and plastocyanin. The reduction of NADP+ by Z– is mediated by soluble ferredoxin and ferredoxin-NADP reductase. It seems possible that the primary acceptor of PS–1, Z, is identical to bound ferredoxin, since the latter is photoreduced at very low temperatures (25°K) (Bearden & Malkin, 1974).
Cytochrome b6 appears to function on a cyclic electron transport pathway around PS–1 and it may play a role in cyclic phosphorylation. There is conflicting evidence concerning the role of cytochrome b– 559HP (Boardman, 1975). This cytochrome is oxidized by PS–2 at liquid nitrogen temperature and under certain conditions at room temperature, but at high light intensity it can also be reduced by PS–2. The function of cytochrome b– 559LP is unknown at present.
Much evidence for the scheme of photosynthetic electron transport depicted in Fig. 4.4 has come from difference spectroscopy of chloroplasts. In this method, the change in the absorbance of various chloroplast components is measured on illumination of the chloroplasts with monochromatic light of various wavelengths. By following the change in the spectrum of individual components e.g. cytochrome ¦ or P–700, the role of these components can be deduced. For example, if chloroplasts are illuminated with far-red light of wavelength 720 nm, which is only absorbed by PS–1, there is a decrease in the absorbance of the chloroplasts in the region of 554 nm, due to the oxidation of cytochrome ¦ . If the chloroplasts are then illuminated with 650 nm light, absorbed by PS–2 (as well as by PS–1)there is an increase in absorbance due to the reduction of cytochrome ¦ . The oxidation of P–700 is followed by a decrease in absorption at 700 nm.
Electron transport from water to NADP+ in isolated chloroplasts may be intercepted by the addition of artificial electron acceptors (oxidants), which accept electrons from Z– in PS–1 or from PQ in PS–2. For example, methyl viologen accepts electrons at PS–1 while p -phenylenediamine intercepts the chain at PQ. Ferricyanide or dichlorophenolindophenol can interact at either PS–1 or PS–2, depending on the experimental conditions (Trebst, 1974).
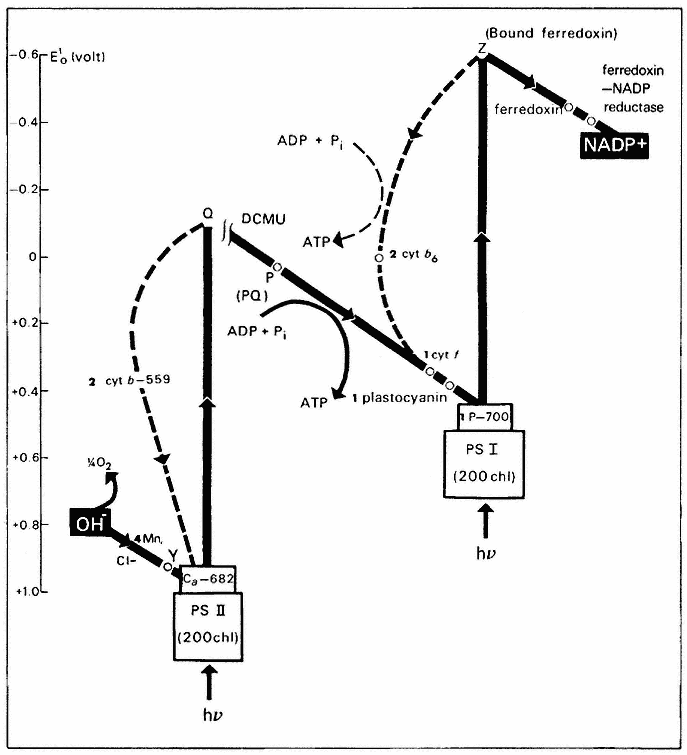
Figure 4.4
Z-scheme for photosynthetic electron transport and photophosphorylation. The
number beside a component indicates the number of molecules of that component per
photosynthetic unit of 400 chlorophyll molecules. Two sites of ATP formation are located
on the pathway between water and PS–1 (see text); one between water and plastoquinone
(PQ) and the other between PQ and PS–1. A scale of redox potentials is shown on the left.
Photosynthetic electron transport is readily monitored by illuminating isolated chloroplasts in the presence of an electron acceptor, and measuring either the oxygen evolved or the amount of acceptor reduced (Hall & Rao, 1972). This reaction is known as the Hill reaction (Hill, 1939).
The herbicides 3(3,4-dichlorophenyl) 1,1-dimethylurea (DCMU) and 3(p -chlorophenyl)-1,-1-dimethylurea (CMU) inhibit electron transport between
Q and PQ. Photoreduction of NADP+ can be restored in the inhibited chloroplasts by the addition of an artificial electron donor such as reduced 2,6-dichlorophenolindophenol. Electrons from the artificial donor enter the electron transport chain between the light reactions, and photoreduction of NADP+ is then driven by PS–1.
PS–1 is known as the far-red system because its absorption spectrum extends to longer wavelengths than that of PS– 2. At wavelengths beyond 700 nm, PS– 1 receives a high fraction of the quanta absorbed by chloroplasts. Chloroplasts contain one molecule of cytochrome ¦ and one molecule of P–700 per 430 chlorophyll molecules, from which it is concluded that the photosynthetic unit contains about 400 chlorophyll molecules. As shown in Fig. 4.4 the chlorophyll molecules appear to be distributed about equally between the two photosystems.
4.4.3—
Photosynthetic Phosphorlation
Electron flow from water to NADP+ is coupled to the formation of ATP. Until recently it was uncertain whether there is one or two energy conserving sites, where ATP is formed. This arose because of the conflicting experimental determinations of the amount of ATP formed during the transfer of 2 electrons from water to NADP (the P/e2 ratio). Earlier measurements gave a P/e2 ratio of one (Arnon et al., 1958), suggesting one energy conserving site, but more recent determinations indicate P/e2 ratios between 1.2 and 1.8 (Trebst, 1974). One energy conserving site is associated with the reoxidation of plastoquinone by cytochrome f, and the second (not shown on Fig. 4.4) is associated with the electron transfer from water to plastoquinone. However, the two sites do not necessarily yield two ATP per 2 electrons transferred from water to NADP+ , as indicated by the experimental P/e2 ratios. A P/e2 ratio of at least 1.5 is needed to provide enough ATP to drive the Calvin cycle, and more ATP is required for the C4 -pathway.
Another type of phosphorylation has been observed with isolated chloroplasts. Known as cyclic phosphorylation, it requires an exogenous cofactor such as phenazine methosulphate, pyocyanin or ferredoxin. Unlike non-cyclic phosphorylation, cyclic phosphorylation is not accompanied by any net change in oxidation or reduction and it is not inhibited by DCMU. The process is driven by light absorbed by PS–1. Chloroplasts exhibit very low rates of cyclic phosphorylation in the absence of an exogenous electron carrier. It is uncertain, therefore, whether cyclic phosphorylation in vivo contributes a significant amount of ATP. Recent work suggests that oxygen may act as an alternative electron acceptor at PS–1 when NADP is fully reduced, and provide extra ATP during electron flow from water to O2 (Heber, 1975). The relative amounts of ATP formed by noncyclic electron flow to NADP+ or O2 could be regulated by the demands of the cell for NADPH and ATP.
4.4.4—
Phosphorylation and Thylakoid Structure
Three main hypotheses have been proposed for the mechanism of ATP formation in mitochondria and chloroplasts (Slater, 1971), but here we will briefly consider only one of these, the Mitchell chemiosmotic hypothesis (Mitchell, 1966; see also chapter 5). The fundamental concept of the chemiosmotic hypothesis is that of vectorial flow of protons across the mitochondrial inner membrane or the thylakoid membrane. Mitchell proposed that the electron transport chains of mitochondria and chloroplasts consist of alternate electron and hydrogen carriers, organized in a vectorial fashion across the membrane. Transport of electrons through the chain of carriers results in the net transfer of protons across the membrane. For the thylakoid membrane, protons are transported from the outside (stroma or matrix) to the inside intrathylakoid space. This is depicted in Fig. 4.5 (Trebst, 1974). In this scheme, reduction of plastoquinone by Q
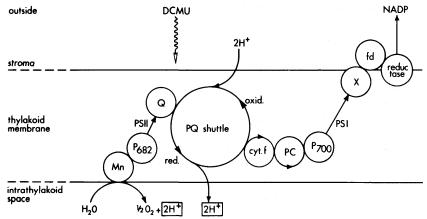
Figure 4.5
Photosynthetic electron flow, showing vectorial arrangement of carriers and the
movement of protons from outside to inside of the thylakoid. (From Trebst, 1974.)
occurs on the outside of the thylakoid and oxidation by cytochrome ¦ on the inside, resulting in the transfer of 2 protons for each 2 electrons transported from water to NADP+ . Water oxidation is considered to take place on the inside to produce 2 protons per 1/2O2 . According to the Mitchell hypothesis, the protons move back through the membrane through special channels which contain the coupling factor or ATPase, and result in formation of ATP from ADP and inorganic phosphate. As already discussed, part of the ATPase (CF1 ) is on the outside of the thylakoid membrane, and part (CF0 ) is embedded in the membrane. It is not yet established whether the proton gradient across the thylakoid membrane is an essential intermediate in the process of ATP formation during photosynthetic electron flow. However, with thylakoid membranes it has been shown
that ATP formation can be driven by a pH gradient created artificially (Jagendorf & Uribe, 1966). Chloroplasts are suspended in a buffer of low pH (pH < 5) and then transferred to a buffer of pH 8, containing ADP and inorganic phosphate.
According to the Mitchell hypothesis, the energy-conserving sites are identified with the proton-releasing sites on the inside of the thylakoid. Substances such as ammonia which uncouple ATP formation from electron transport in the thylakoid membrane (termed uncouplers) are considered to destroy the proton gradient by transporting the protons across the membrane.