Magma Chambers
Volcanic products are generally classified by their major-element chemistry (Fig. 1.2) or their modal phenocryst content (Fig. 1.3). These classification schemes (Appendix D) are useful in relating volcanic rocks to magma types. Accordingly, the origin and evolution of magma types can be interpreted in a general manner by considering igneous compositional trends: tholeiitic, transitional, alkalic, potassic, and calcalkalic (Carmichael et al ., 1974). When the field geologist examines pyroclastic samples that do not lend themselves to the above classification schemes, the color of glass shards can be simply related to their refractive index as a function of silica content (see Appendix D as well as Williams et al ., 1982, p. 73). Rock classification has been a traditional exercise for volcanologists, and today the results of this work can be used to determine the nature of the magma source: its shape, depth, and longevity—all of which are important components when evaluating geothermal potential.
One of the most significant recent advances in volcanology is the development of a system for relating the chemical aspects of volcanic rocks to magma-chamber dynamics. This effort has been most fruitful in the cases of volcanoes that have developed calderas (Williams, 1941). Smith (1979) shows a direct correlation between caldera area and the volume of products expelled during the caldera-forming eruption (Fig. 1.4). Where caldera eruptions produce ash flows of differentiated products, Smith and Shaw (1975, 1979) and Smith (1979) show that the volume of these products is ~10% of the volume of the underlying magma chamber. This simple concept has profound implications in the search for geothermal heat sources because by using eruption age constraints, cooling models can predict the residual heat left in and around the magma chamber (Fig. 1.5). Furthermore, there is growing evidence that zonation of magma chamber chemistry can be documented by analyses of time-series chemical trends in eruption products (for example, Hildreth 1979; 1981).
Nonbasaltic volcanic rocks are considered to be products of evolved magmas. Hildreth (1981) stated, "every large eruption of nonbasaltic magma taps a magma reservoir that is thermally and compositionally zoned," and "most small eruptions also tap parts of heterogeneous and evolving magmatic systems." One general hypothesis is that evolved or otherwise differentiated magmas have a crustal reservoir. The volume of a crustal magma chamber is directly proportional to the time required for it to evolve. Consequently,
|
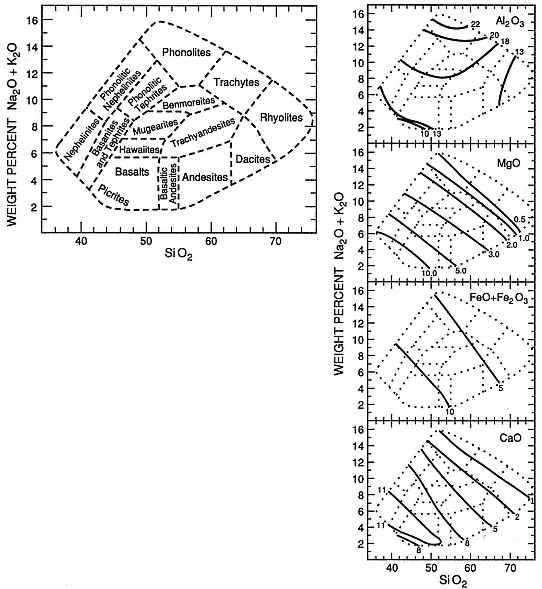
Fig. 1.2
Classification of volcanic rocks by major-element chemistry as expressed in alkali-silica variation
diagrams; details for major oxide concentrations (at right) are shown weight percent.
(Adapted from Cox et al ., 1979.)
differentiated volcanic products—especially where they are several cubic kilometers in volume—are good indicators of a crustal magma chamber (Fig. 1.5).
Smith's (1979) observation of the correlation between caldera area and ejecta volume opened the door for interpretation of chemical zonation in silicic magma chambers. He predicted that "all caldera-forming ash-flow sheets should, when studied in detail, show some degree of chemical and/or mineralogic gradients inherited from the magma chamber." Hildreth (1979) documented such gradients in the Bishop Tuff in eastern California. Assuming that earlier erupted products originate from the top of a magma chamber and later materials derive from lower portions, it is likely that the time-sequenced chemical characteristics of
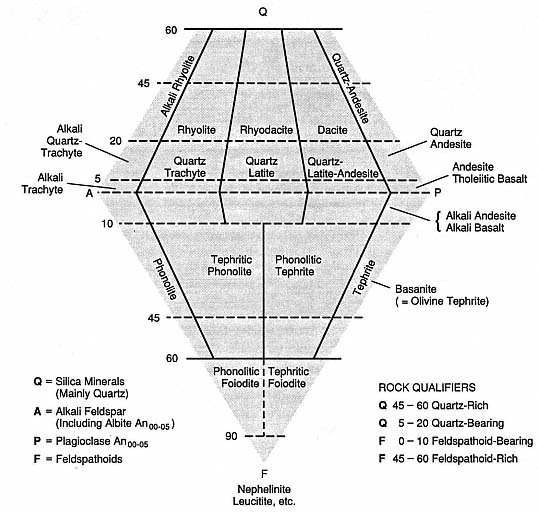
Fig. 1.3
Classification of volcanic rocks by modal phenocryst content plotted on a quartz (Q) - alkali feldspar
(A) - feldspathoid (F) - plagioclase (P) diagram. Petrographic analysis may not always be sufficient to
determine the percentage of minerals present in volcanic rocks; a calculated mineral composition,
based upon normative (chemical) composition might be necessary. Williams et al . (1982) question
this approach to classification because it presupposes "the need to agree upon a single rational and
workable system . . ." and it incorporates both "igneous and igneous-looking rocks" in such a
way that it may conceal the natural association and relative abundances of rock types.
(Adapted from Streckheisen, 1967.)
volcanic ejecta depict an inverse order of the magma chamber's compositional stratification (Fig. 1.6). This chemical stratification also is reflected by oxygen fugacity and mineral equilibrium temperatures that increase with time in products from a large eruption (Hildreth, 1981). Several other petrologic features of volcanic ejecta that suggest magma chamber zonation are isotopic ratios, phenocryst abundances that increase with SiO2 values (Fig. 1.7), and volatile component abundances. This latter feature is best typified by stratigraphic relationships showing that early products resulted from more explosive, gas-rich eruptions and later materials were from

Fig. 1.4
Correlation between caldera area and volume of products (ash flows); diagonal lines plot the
model's draw-down depths of magma chambers.
(Adapted from Smith, 1979.)
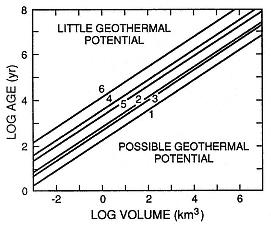
Fig. 1.5
Conductive models of heat resource as a function of the age and volume of magma
chambers. Odd numbers refer to slab-like magma chamber shapes and even numbers
represent cubic shapes. Lines 1 and 2 take into account heat transfer effects of con-
vection within the magma body, whereas lines 3 and 4 ignore this effect. Systems in
which estimated magma bodies plot above line 5 and 6 have cooled to near ambient
temperatures; those plotting below lines 1 and 2 may still have near solidus tempera-
tures; and those plotting between lines 3 and 4 are at post magmatic temperatures
>300°C. (Adapted from Smith and Shaw, 1975.)
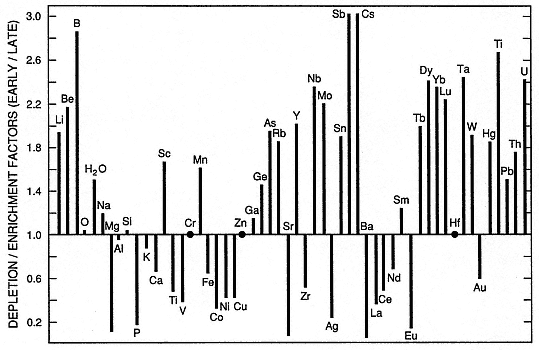
Fig. 1.6
Plot of elemental enrichment factors as abundance ratios of early to late products of the Bishop
Tuff eruptions shows the overall difference in magma composition. The line drawn at an
enrichment factor of 1 demarks no enrichment or depletion; elements plotting above this line are
enriched in early products and those plotting below the line are enriched in late products.
These enrichment factors are interpreted to reflect magma chamber zonation, assuming
that the eruption taps different parts of the chamber with time.
(Adapted from Hildreth, 1981.)
gas-poor effusive extrusion. However, Eichelberger et al . (1986) suggested that this apparent volatile zonation in rhyolitic eruptions might only reflect eruptive conditions. In Eichelberger's model, the volatiles are not stratified in the magma. Early eruptions are explosive because the volatile flux is confined within a narrow vent region, whereas later effusive eruptions involve a gradual degassing of the rhyolite through permeable vent-wall rocks—a process that results in a volatile-poor magma by the time it reaches the surface and is extruded as a lava flow.
Although major-element abundances do support hypotheses of magma-chamber zonation, it is analyses of the trace elements that best portray the nature of the zonation and mechanisms of differentiation as a result of their variable compatibility in various phenocryst and liquid phases (Fig. 1.8). Petrologic studies of magma suggest that large chambers are fundamentally basaltic because mantle melting supplies heat to the crust for crustal melting, provides a mafic component to hybridize with the crustal melts, and generates a thermal gradient to drive various differentiation processes in the crustal magma reservoir (Fig. 1.9). This general evolution of crustal magma chambers may depend upon tectonic environment (Fig. 1.10).
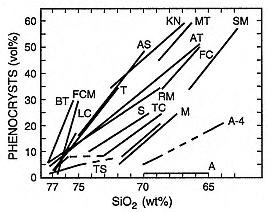
Fig. 1.7
Plot of phenocryst abundances vs bulk-rock SiO2 of silicic magma for various
volcanoes. The decrease of phenocryst abundance with increasing SiO2 content is
interpreted to reflect liquidus depression caused by dissolved volatiles, which are
supposed to be more abundant in silica-rich magmas found near the tops of magma
chambers. A = caldera-forming eruption of Aniakchak (Miller and Smith, 1977);
FC = Fish Canyon Tuff (Lipman, 1975); FCM = Fish Creek Mountain Tuff (McKee,
1970); KN = Kneeling Nun Tuff (Elston et al ., 1976); MT = Monotony Tuff (Ekren et al ., 1971);
LC = Lava Creek Tuff (Christiansen and Blank, 1972); SM = Snowshoe Mountain Tuff (Ratté
and Steven, 1967); AS = Apache Springs Tuff (Rhodes, 1976); AT = Ammonia Tanks
(Byers et al ., 1976); A-4 = Aso-4 (Lipman, 1967); BT = Bishop Tuff (Hildreth, 1979);
RM = Ranier Mesa (Christiansen et al ., 1977b); S = Shikotsu caldera eruption
(Katsui, 1963); T = Tshirege (Smith and Bailey, 1966); TC = Tiva Canyon
(Christiansen et al ., 1977b); TS = Topopah Spring (Christiansen et al ., 1977b).
(Adapted from Hildreth, 1981.)