3.2—
Chloroplast Structure
3.2.1—
Chloroplast Dimensions and Number
Beans possess chloroplasts typical of the sun leaves of higher plants in that they are discoid or lens-shaped with a diameter of about 5µm and maximum thickness of about 1 µm as may be seen in the electron micrograph in Fig. 3.1B, which is a vertical section through the disc. On the other hand observations of chloroplasts in living cells by phase contrast microscopy suggest that the maximum thickness of the chloroplast may be rather less (S. G. Wildman, personal communication). Under optimum conditions for growth, Phaseolus vulgaris averages 45 chloroplasts per palisade mesophyll cell and 32 per spongy mesophyll cell, giving about 8.3 × 108 chloroplasts per leaf and about 2.3 × 107 chloroplasts per cm2 of leaf. This latter number is similar to that of mature spinach leaves (Possingham & Saurer, 1969) although the number of chloroplasts per spinach mesophyll cell is more than ten times greater than for bean.
3.2.2—
Chloroplast Fine-Structure
The following interpretation of chloroplast fine structure is based on transmission electron microscopy of thin sections of leaf material prefixed in 3% glutaraldehyde for 24 hours at 5°C, followed by fixation and staining with osmium tetroxide and post-staining with uranyl acetate and lead citrate. This technique is the most widely used, and, as glutaraldehyde is a relatively mild reagent, is considered to produce images most closely resembling the living plastid. Heslop-Harrison (1966) has written a critical account of the interpretation of chloroplast electron micrographs while the results of freeze-etch studies are considered in chapter 4.
The chloroplast envelope consists of two membranes (Figs. 3.1 and 3.2) the outer of which is unspecifically permeable to crystalloidal solutes. In contrast the inner membrane shows very specific permeability and has so far been shown to be the site of three specific anion translocation systems; (a) the phosphate translocator, facilitating a counter exchange of inorganic phosphate, 3-phosphoglycerate and dihydroxyacetone phosphate; (b) the dicarboxylate translocator, facilitating a counter exchange of dicarboxylic acids; and (c) the ATP translocator which is less active than the previous two and may be responsible for the entry of ATP in the dark (Heldt et al., 1972).
Within the inner membrane is a complex system of flattened sacs of membrane which were first termed thylakoids by Menke (1962). The thylakoids are closely associated in stacks (grana) which are shown in section for chloroplasts of bean and maize mesophyll in Fig. 3.1 (B and C). When seen from above the plane of the membrane the grana are essentially circular in outline with an average diameter of about 0.5 µm. Under optimum growing conditions bean grana
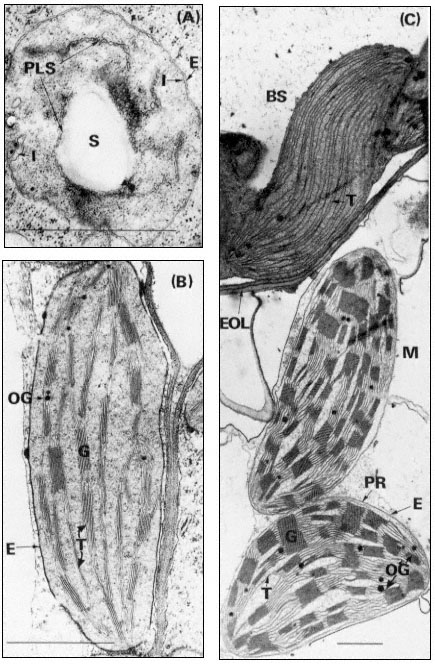
Figure 3.1
Plastids of bean and maize. A, proplastid from the primary leaf of a six day-old dark-grown
bean (× 42,000); B, chloroplast from the primary leaf of a bean grown for 14 days in the dark
and then transferred to continuous illumination (3 mW. cm–2 ) for 48 hours (× 29,000); C, bundle
sheath and mesophyll chloroplasts of maize grown under normal diurnal illumination (×12,500).
Scale lines represent 1 µm. Key to lettering: BS, bundle sheath; E, plastid envelope; EOL, electron
opaque layer; G, granum; I, invagination of envelope inner membrane; M, mesophyll; OG, osmiophilic
globule; PLS, porous lamellar sheet; PR, peripheral reticulum; S, starch grain; T, thylakoid.
contain up to 8 thylakoids while those of maize have up to 40. The thylakoids in each granum are continuous with those in other grana through intergranal thylakoids. In bean the granal thylakoids average 78%, and the intergranal thylakoids 22%, of the total thylakoid membrane (Bradbeer et al., 1974a). During chloroplast development the inner membrane of the envelope appears to give rise to the thylakoids by invagination (see Fig. 3.1A and p. 75) and it is possible that the space between the inner and outer membranes of the envelope is continuous with the whole of the thylakoid space of the chloroplast. Although the magnification of Figs 3.1B and C is such as to allow an overall impression of chloroplast structure, enough detail is visible to show that the intra-thylakoid space is electron-transparent, the thylakoid membrane is electron-opaque and the inter-thylakoid space seen in the grana between adjacent thylakoids is densely electron-opaque.
In the chloroplast the thylakoids are embedded, or suspended, in a matrix, the stroma, which has a somewhat granular appearance (Fig. 3.1). Within the stroma may be seen DNA fibrils and ribosomes (chapter 9), starch grains, osmiophilic globules and occasional extensive crystal-like structures. Such crystals can often be induced to appear in chloroplasts suspended in hypertonic media or in plants subjected to stress, although they have also been observed in plants grown under normal environmental conditions. It is thought likely that such crystals are composed of ribulosebisphosphate carboxylase (Larsson et al., 1973).
3.2.3—
The Mobile and Stationary Phases of Chloroplasts
From observations of chloroplasts in living cells by phase-contrast microscopy Wildman (1967) distinguished two components of the chloroplasts, a stationary phase which he equated to the thylakoid system and a mobile phase which he equated to the stroma. The mobile phase surrounds the grana and also penetrates the intergranal regions of the chloroplast. The mobile phase is always in some kind of motion though the intensity of the activity shows variability, even in the same chloroplast. In the reported investigations, observations were always made in cells which could be seen to be living by the presence of visible protoplasmic streaming. In such cells the mobile phase formed protuberances which sometimes broke away from the chloroplast and became indistinguishable from
mitochondria, and also mitochondria-like bodies appeared to fuse with the mobile phase. Subsequently Wildman and coworkers (1974) have reported that mitochondria-like bodies became stationary below tobacco chloroplasts in living cells and that starch grains subsequently appeared in similar positions in the chloroplasts. In the opinion of the present writer the interpretation that mitochondria give rise to starch grains cannot be substantiated. We have found that in plants grown under low light intensities each chloroplast has one or more mitochondria embedded in deep pockets close to the chloroplast margin but that no break in the chloroplast envelope occurs and there is always at least a thin layer of cytoplasm between chloroplast and mitochondrion (Montes & Bradbeer, 1976). It is possible that some of the observations of Wildman and colleagues may be explained by the development of the latter phenomenon.
3.2.4—
The Range of Chloroplast Structure
Quite early in the application of electron microscopy to plant structure the algae were found to show an interesting range of structural diversity of their chloroplasts. Subsequent studies on vascular plants established that a number of structural types were to be found in both cultivated and non-cultivated plants and furthermore that structural modification might be induced by mutation or by variation of the environmental conditions.
3.2.4.1—
The Algae
A monograph such as that of Dodge (1973) should be consulted for details of the range of chloroplast structure of this group. The characteristics of the chloroplasts have provided an important part of the basis for the taxonomic classification of the algae. In the green algae (Chlorophyceae and Prasinophyceae) the arrangement of the thylakoids tends to be basically similar to that in vascular plants and many of the differences may be associated with the non-discoid shape of the chloroplasts in most green algae. The simplest arrangement is found in the Rhodophyceae where the thylakoids occur as single sheets. In the Cryptophyceae the thylakoids tend to occur as pairs but the individual thylakoids in the pair do not appear to be fused to each other. The other algal classes have their thylakoids arranged in threes; in some cases (Dinophyceae, Euglenophyceae and Haptophyceae) the component thylakoids appear to be fused to each other while in other cases (Chrysophyceae and Phaeophyceae) the thylakoids do not appear to be fused. In two algal classes (Dinophyceae and Euglenophyceae) the chloroplast envelope consists of three membranes instead of two. In a number of classes (Bacillariophyceae, Chloromonadophyceae, Chrysophyceae, Cryptophyceae, Haptophyceae, Phaeophyceae and Xanthophyceae) the chloroplast is also surrounded by a sheath of endoplasmic reticulum which is usually continuous with the nuclear membrane. To complete this brief list of
the major peculiarities of algal chloroplasts it should be noted that the chloroplasts of many algae possess pyrenoids and eye spots.
3.2.4.2—
The Dimorphic Chloroplasts of C4 Plants
C4 plants form oxalacetate, malate and aspartate as the primary products of their photosynthetic CO2 -fixation in contrast to the more 'usual' C3 plants whose primary fixation product is 3-phosphoglycerate. Laetsch (1974) lists the following families of flowering plants as containing C4 species: Amaranthaceae, Aizoaceae, Chenopodiaceae, Compositae, Cyperaceae, Euphorbiaceae, Gramineae, Nyctaginaceae, Portulaceae and Zygophyllaceae. All of these families also contain C3 plants and there is the case of the genus Atriplex where the C4A. rosea will hybridize with the C3A. patula (Björkman et al., 1970). Apart from the difference in primary photosynthetic CO2 -fixation products C4 and C3 plants show other substantial differences in their biochemistry, physiology, anatomy and fine structure. Maize and bean will be discussed here as typical examples of C4 and C3 plants respectively
In the maize leaf the chloroplasts are concentrated in two concentric sheaths of cells around each vascular bundle. The inner sheath of cells is described as the bundle sheath and consists of equal numbers of large barrel-shaped cells and smaller cells which can be divided into two sorts on the basis of their dimensions (Montes & Bradbeer, 1975). Laetsch (1974) points out that the thick walls of bundle sheath cells adjoining mesophyll cells in C4 grasses possess an electronopaque layer (Fig. 3.1C). The bundle sheath chloroplasts possess abundant thylakoids which do not associate into grana, (Fig. 3.1C) and they normally contain starch grains. The plant from which the material was taken for Figure 3.1C had been stored in the dark for 24 hours prior to fixation to remove the starch so as to obtain a clear electron micrograph. In most cases, the bundle sheath chloroplasts of maize have been found to be completely agranal, although by modification of the environmental conditions the formation of grana can be induced (Bradbeer & Montes, 1976). In contrast Laetsch (1974) considers it normal for bundle sheath chloroplasts to show a small amount of thylakoid appression.
The outer sheath of cells is known as the mesophyll sheath and it contains chloroplasts similar to those of C3 plants in that they have grana. They are, however, different in that they do not normally contain starch grains. Not all C4 plants show such structural dimorphism of their chloroplasts. However all chloroplasts of C4 plants possess a system of tubules, the peripheral reticulum, (PR in Fig. 3.1C), which is associated with the inner membrane of the chloroplast envelope. There are reports of peripheral-reticulum-like membrane systems in chloroplasts of some cells of C3 plants (Laetsch, 1974). Circumstantial evidence assembled by the latter author suggests that the function of the peripheral membrane in C4 plants may be to facilitate transfer of metabolites between chloroplast and cytoplasm.
3.2.4.3—
The Effect of Environmental Conditions on Chloroplast Structure
The fact that plants grown in the complete absence of illumination form etioplasts (Fig. 3.2A and section 3.4.2) while those grown under diurnal illumination form chloroplasts establishes that light is an essential requirement for chloroplast development. When Björkman et al., (1972) compared plants of Atriplex patula which had been grown under three different irradiances: 20, 6.3 and 2 mW. cm–2 (in the waveband 400–700 nm), which are referred to as high, intermediate and low, they found that the high irradiance treatment yielded thicker leaves than the low, with more cells per leaf section and more chloroplasts per cell. The chloroplasts from plants grown under low irradiances were larger than those from high irradiance conditions and they contained more thylakoids and larger grana. The intermediate illumination gave intermediate results. The low irradiance-grown Atriplex plants were compared with plants adapted for growth on the floor of the Queensland rainforest where the daily quantum flux was about one-twentieth of that provided by the low irradiance treatment. The chloroplasts of these plants, Alocasia macrorrhiza, Cordyline rubra and Lomandra longifolia were found to be dark green, unusually large and irregular in outline and to contain very well developed grana of enormous size (Anderson et al., 1973). The grana were also irregularly arranged and apparently adapted for a maximum efficiency in light-trapping.
When maize plants were grown under very low irradiances (0.3 mW. cm–2 ), which nevertheless provided about six times the daily quantum flux received by the rainforest plants, some chlorophyll and photosynthetic CO2 -fixation developed even though the light compensation point was not exceeded and the leaves showed a net loss of CO2 followed by senescence and premature death (Bradbeer & Montes, 1976). Grana did not develop and both bundle sheath and mesophyll chloroplasts developed closely parallel arrangements of thylakoids which did not become appressed. Transfer of plants greened under very low irradiances to higher irradiances resulted in a partial recovery towards normal structure.
Research work in Belgium has shown that exposure of etiolated seedlings to electronic flashes of 1 millisecond in duration and given at 15 minute intervals brought about greening in which the resultant plastids were agranal with parallel unfused thylakoids. These flashed leaves showed the interesting property that, on transfer to continuous illumination, they initially did not show any photosynthetic oxygen evolution, but within 2 minutes of the commencement of illumination oxygen evolution was detected and a maximum rate was found after 6 minutes (Strasser & Sironval, 1972). When etiolated leaves are transferred to continuous illumination without any pretreatment with light the onset of oxygen evolution tends to be considerably delayed. An alternative treatment which has produced almost completely agranal plastids is exposure of dark-grown seedlings to continuous far-red irradiation at wavelengths longer than 700 nm (De Greef et al., 1971). For further discussion see page 80.
3.2.4.4—
Chloroplast Mutants
Many chloroplast mutants are known with defects in pigments or other components of structure or function. Some of the mutations are nuclear and show normal Mendelian inheritance whilst others show non-Mendelian inheritance and are considered to be mutations of the chloroplast DNA. Chloroplast mutations in algae can be maintained in heterotrophic culture and have been used for important research on chloroplast genetics, development and function (see e.g. Levine, 1969). In contrast, although there have been numerous investigations of individual chloroplast mutations in higher plants, the only substantial research collection of higher plant material with mutant chloroplasts is the barley mutant collection of D. von Wettstein's group in Copenhagen (von Wettstein et al., 1971). This collection has so far not been made generally available for studies on chloroplast development.