2.3—
Membrane Structure
It is convenient to discuss membrane structure under two headings; the organization of the membrane matrix which is largely a matter of the relationships of the lipid components, and the substructure of the protein in the membrane.
2.3.1—
The Membrane Matrix
2.3.1.1—
Phospholipids
The structure of phospholipid molecules considered earlier provides the key to understanding why it is that the membranes as seen in transverse sections have
a characteristic trilaminar appearance. The hydrophilic head regions make hydrogen bonds with water and may become cross-linked to other heads and to proteins through ionic bridges, e.g. by calcium ions; thus they are organized into a lattice-like structure. By contrast the long acyl chains of the two fatty acids attached to each phospholipid are strongly hydrophobic, loosely organized and, above their melting point, are relatively fluid. If phospholipid is dispersed in water the 'tails' will take on a conformation which will minimize their contacts with water. The 'heads' will, of course, react favourably with water. If the available water surface is large relative to the amount of lipid, the molecules will arrange themselves as a film-like monolayer with the heads at the water surface and the 'tails' protruding from it at right angles (Fig. 2.6a). If more phospholipid molecules are added to this system so that there are more than can be fitted into a tightly packed monolayer over the water surface, a second type of arrangement occurs quite spontaneously. The phospholipids form two ranks with the heads facing outwards in both and the tails directed inwards to form a non-polar hydrophobic layer sandwiched between them (Fig. 2.6b). This bilayer arrangement, which is common to all biological membranes, can also be formed from mixtures of phospholipids under laboratory conditions. The synthetic membranes thus produced have helped in arriving at an understanding of many of the structure/function relationships of natural membranes (see Goldup et al., 1970, for a readable review).
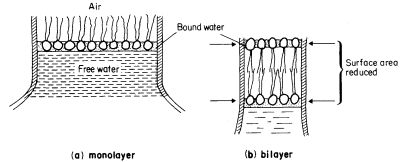
Figure 2.6
An illustration of how a monolayer of dispersed phospholipid (a) in water, forms
into a bilayer, (b) on contraction of the water surface area. The phospholipid
heads have water bound to them in polarized multilayers (see p. 35).
The selected analyses in Table 2.2 show that a given membrane may contain several types of phospholipid as well as appreciable quantities of sterol. It is probable that there is a great deal more organization of phospholipids in natural membranes than can be demonstrated positively at present. Lipids of one kind may be associated into clumps so that the membrane surface may be very heterogeneous with lipids of differing physical properties arranged in a mosaic.
A mosaic of charged and uncharged areas might occur because some phospholipids carry a net electrostatic charge at normal pH values, e.g. phosphatidyl glycerol, while others, like phosphatidyl choline (lecithin) are neutral. This is of significance because it has been shown that, in synthetic bilayers, the surface charge on the phospholipid heads can partly determine both the ion-selectivity and the cation permeability of the membrane (Papahadjopoulos, 1971), and it may also be relevant in determining regions of the surface of the plasmalemma where endo-cytosis may occur (see p. 61).
Local variations in the packing of sterols may render some parts of the membrane less fluid than others and thus determine areas where diffusion may be severely restricted (Papahadjopoulos et al., 1973).
More recently researchers have begun to investigate the possibility that the inner and outer halves of the bilayer may differ in their phospholipid composition. Should this prove to be the case, then it is possible that the barrier properties of the membrane to solute diffusion may be different when the membrane is approached from different sides.
In some special circumstances phospholipid molecules may become arranged into globular micelles in which the polar groups are directed towards the periphery of the sphere, the surface being hydrophilic. This state of affairs can be induced by dehydration in synthetic membrane systems, and in nature by viruses which create membrane instability, e.g. sendai virus, and by certain phospholipids (e.g. lysolecithin) with wedge-shaped head regions which tend to induce curvature of layers of closely packed phospholipids when they are introduced into a bilayer (Lucy, 1970). It has been suggested that rapid local transitions from the predominant bilayer to the micellar state are important in membrane fusion and in pinocytosis (see Lucy, 1970). If these transitions do occur then it is possible that they may cause transient gaps or pores to be created in the membrane; much physiological evidence points to the conclusion that membranes do have very fine pores in them (see p. 59).
2.3.1.2—
Sterols
The insertion of sterol molecules into the membrane increases the structural order of the hydrophobic region. These molecules lie with their long axes parallel to the hydrocarbon chains of the fatty acids with their more rigid ring structures directed towards the outside and their open chain ends towards the centre. The mobility of the hydrocarbon chains nearest to the outside of the membrane is, therefore, restricted by these stiffening structures but they remain pliant at their ends so that the central region is fluid (Caspar & Kirschner, 1971). The rigidity conferred on the membrane by the inclusion of sterols slows down the diffusion of materials through the outer part of the lipid domain in synthetic bilayers (Papahadjopculos et al., 1973).
2.3.1.3—
A Model of the Membrane Matrix
Figure 2.7 provides a basic interpretation of the ideas on the membrane matrix discussed so far. A fact, which it is important to understand but which is difficult to illustrate, is that the centre of the membrane is fluid while the periphery is semi-crystalline. Although it is a stable structure, it is known that phospholipid molecules can be inserted into, and withdrawn from the matrix rapidly and that the structure illustrated in Fig 2.7 represents a dynamic steady state when it is part of a biological membrane.
2.3.2—
Membrane Sub-Structure
When a cell or a piece of tissue is frozen and then fractured with a suitable blade, the fracture plane will follow lines of weakness in the structure. Since the central region of the membrane matrix contains no ice it is a potential line of weakness, along which the membrane tends to fracture (Fig. 2.8). Where the fracture line passes tangentially across a cell or organelle, sheets of membrane material become apparent; since membranes tend to be cleaved down the middle it is obvious that the surface exposed is not the true membrane surface but is the membrane interior. Figure 2.9 shows a relatively smooth sheet of plasmalemma from an onion root tip on which numerous round particles and depressions can be seen. Some of the particles are arranged in files while others are randomly distributed. These particles, which are usually 6–9 nm in diameter, are embedded in the membrane and are not resting on its true surface. This was demonstrated by Pinto da Silva & Branton (1970) who etched away the ice from the fracture plane by leaving the specimens under a high vacuum for some minutes after fracturing them. Using this method, the true surface of membranes which lay obliquely to the fracture plane was eventually revealed as the ice from the surrounding cytoplasm sublimed. The true surface had a much smoother appearance, the undulations of which gave the impression of a blanket lying over the embedded particles. The particles in the membrane can be removed by treatment with proteolytic enzymes and can be re-created in synthetic membranes which have been made in the presence of a hydrophobic protein. Vail et al., (1974) reported that a synthetic bilayer membrane into which a hydrophobic protein had been incorporated had intercalated particles of 8.5 to 9 nm diameter occupying 12% of the internal membrane surface whereas in bilayers lacking the protein there were none.
The frequency of particles varies according to membrane type (Table 2.5); chloroplast and mitochondrial membranes have the highest frequency as one might anticipate from the many metabolic events which are centred upon them. One of the two fracture faces is usually more densely populated than the other. Bearing in mind the amphipathic nature of membrane proteins (see p. 34) it is possible that the more densely populated face may reflect the principal orientation of the polar groups of the integral proteins, i.e. if most of the polar groups were directed towards the outside of the cell or compartment, the outer fracture
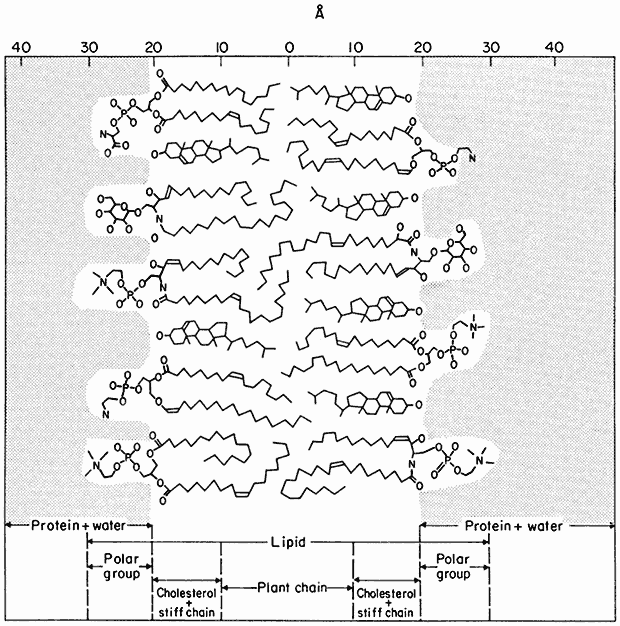
Figure 2.7
Orientation of polar lipids, cholesterol and peripheral protein in a model of the membrane matrix.
Based on X-ray diffraction analysis of nerve myelin. (From Caspar & Kirschner, 1971). In many
ways myelin has been an unfortunate choice for detailed structure of cell membranes since it is
not typical in having virtually no integral protein and has no intercalated membrane particles
(see Table 2.5). It is, however, very appropriate as a general model of the membrane matrix.
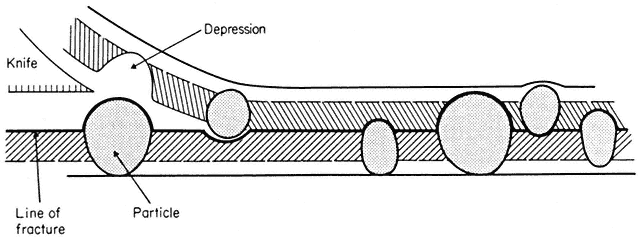
Figure 2.8
Line of fracture when a frozen membrane is split open in freeze-fracturing. Particles
embedded in the membrane become apparent in the cleavage plane either as
projections or as hollows if they are removed on the upper half of the bilayer.
face would be the most densely populated. It should be noted that in synthetic lecithin membrane and in nerve myelin, which both have a very low permeability to ions, there are no particles.
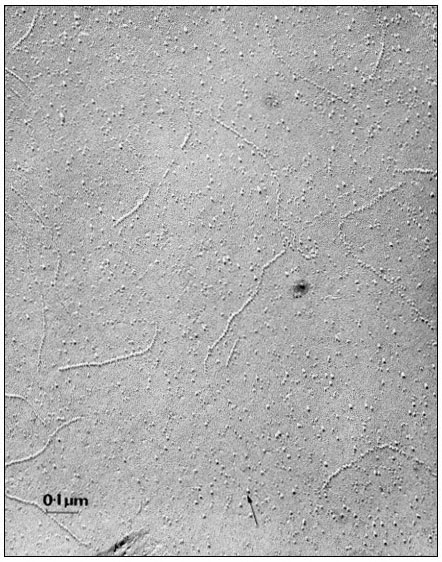
Figure 2.9
Particles seen on the inner fracture face of the plasmalemma from a root tip
cell in onion. The fracture face exposed as described in Fig. 2.8 and metal
shadowed to produce a replica; the arrow represents the direction of
shadowing. Note that there are distinct files of particles as well as randomly
distributed individuals. The membrane matrix appears to be relatively smooth.
(Micrograph by courtesy of Professor D. Branton).
|
2.3.2.1—
A Model of Membrane Structure
The representation of membrane structure shown in Fig. 2.10 is one which commands wide acceptance at the present time. In it we see that large masses of integral protein are inserted to varying extents in the membrane matrix while some are restricted to the surface and others protrude right across the width having a dumbell configuration. The model, which has become known as the fluid mosaic (Singer & Nicholson, 1972), has a disorderly appearance in contrast to the neat pictures used to illustrate the unit membrane; the membrane sub-structure has been likened to numerous protein icebergs in a sea of lipid.
2.3.2.2—
Membrane Fluidity
An essential feature of the model in Fig. 2.10 is that the various components of the membrane can move relative to one another. Rather than being fixed points, many of the proteins are best thought of as drifting around in the lipid, the viscosity of which will determine the rate at which they move. Evidence that membrane particles can move in the plane of the membrane comes from freeze
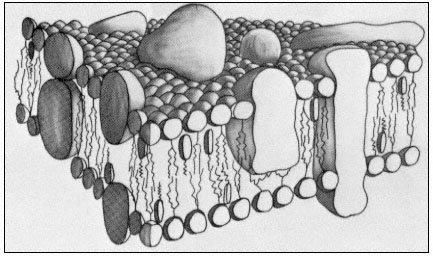
Figure 2.10
A representation of the fluid mosaic model of membrane structure
showing peripheral and integral proteins in a 'sea' of lipid molecules.
Not shown are the polarized water layers which are bound to the polar
regions of the lipids and proteins. The round-headed objects with twin
tails represent phospholipids, the obovoid structure with the single tail
represents sterol and the large irregularly shaped objects are proteins.
(Based on ideas in Singer & Nicholson, 1972 and Capaldi, 1974).
fracture studies of the plasmalemma of Mycoplasma mycoides, in which it was seen that particles became aggregated as the membrane was cooled down but dispersed again when it was warmed up (Rottem et al., 1973). A second line of the evidence comes from studies where two animal cells were induced to fuse with one another (Edidin & Farnbrough, 1973). One of the cells has a specific antigen in its plasma membrane which the other cell lacked. The distribution of the antigen could be visualized by the binding of a fluorescent antibody. The fusion of the cells to form a heterocaryon and the subsequent redistribution of the fluorescent antibody were observed using a fluorescence microscope. At first the fluorescent marker was restricted to one half of the heterocaryon, but quite quickly, and in an orderly progression the fluorescence spread right around the coat indicating that the antigenic protein, known to be integrated into the membrane matrix, had diffused in the plane of the membrane. For it to have done this it must have drifted through the lipid. If the heterocaryon was cooled below the transition temperature of its lipids, no mixing of the antigen occurred.
From what we have said above and from earlier comments (p. 29) it is clear that temperature will have a very important influence on the diffusion of materials through, and in the plane of, the membrane because of its effect on the viscosity of the lipid (Edidin, 1974). If the membrane is cooled to the extent that the lipids freeze then such diffusion will become very restricted; the biological
significance of this is reflected in the fact that the rates of most physiological processes examined over a range of temperatures are found to have a sharp transition at a temperature which is close to the transition of membrane lipids from a liquid crystalline condition to the gelled condition (Simon, 1974).
Most workers agree that there must be some proteins whose position in the membrane relative to others must be maintained, e.g. components of electrontransport chains. Such proteins may require anchoring points either in parts of the membrane which are less fluid than others, or by association with some extra-membrane protein. It is possible that aggregations of sterol molecules might serve to create more viscous patches, and there is evidence, again from synthetic lipid bilayers, that cholesterol can be concentrated in association with certain phospholipids (de Kruyff et al., 1974).
2.3.2.3—
Membrane Synthesis and Flow
The intermediary metabolism concerned with the synthesis of the components of the membrane is beyond the scope of this chapter but it is believed that the components themselves may be centrally assembled and then distributed to the various membranes of the cell. This flow of membrane material can be detected in experiments where cells are provided with a radioactive precursor to a common membrane protein for a short while, and then returned to non-radio-active medium. This type of analysis has not been performed on plant cells but in animal cells, harvested at intervals after pulse-labelling, the radioactive label appears first in the endoplasmic reticulum and then in the Golgi cisternae. Subsequently, the radioactivity of these compartments declines followed by an increase in label associated with the plasmamembrane some hours later (see Table 2.6). Since mitochondria and chloroplasts probably do not have all of the metabolic apparatus to assemble their own membranes it is thought that they too may obtain partly finished membranes from the endoplasmic reticulum (see also chapter 8).
|
There is evidence that the cytoplasm contains numerous membrane-bounded vesicles which frequently appear to be in conjunction with the major membranes
of the cell (e.g. Mahlberg et al., 1974). They are particularly prominent in cells synthesizing walls and, since it is known that precursors of wall synthesis are formed inside the cell and elaborated outside, it is reasonable to conclude that the vesicles contain precursors which are discharged after fusion with the plasmalemma (Heyn, 1971). It has also been found that particulate material from the external medium can be detected in vesicles within the cytoplasm (Mayo & Cocking, 1969; Robards & Robb, 1974). These results suggest that vesicles can both fuse with, and be formed from the plasmalemma and other membranes, thus allowing materials to pass out of or into the cell without their having to cross a membrane (see p. 61); such movements are known as exoand endo-cytosis, respectively. Membrane fusion also presents opportunities for the transfer of blocks of membrane from place to place as is implicit in the observations in Table 2.6.
Since adjacent membranes can frequently be found to be in contact over comparatively large areas and yet show no tendency to fuse with one another, it is believed that special proteins or phospholipids (e.g. lysolecithin) in the vesicle membrane may trigger fusion where they make contact with the larger membrane sheet (see Lucy, 1970).