Chapter 14—
Phytochrome Action
14.1—
Introduction
Light, in addition to providing the energy which drives photosynthesis, also acts as a regulatory environmental stimulus. Many light-controlled plant responses are now believed to be mediated by the photoreceptor, phytochrome. This pigment has a regulatory role in all phases of plant growth and development (photomorphogenesis) and is apparently ubiquitous in all taxonomic groups of eukaryotic plants with the exception of fungi.
The central question of the molecular mechanism of phytochrome action is, however, yet to be resolved. Approaches to this problem fall into two broad categories: (a) Examination of the physical and chemical properties, localization and behaviour of the phytochrome molecule itself; (b) Investigations of the diverse array of phytochrome-mediated plant responses demonstrable at almost any level from the molecular to the gross morphogenetic. The principal findings and concepts that have emerged from such studies are summarized next. Some of the evidence that has led to these concepts is examined in subsequent sections.
14.2—
Phytochrome Dogma
Phytochrome is a blue-green biliprotein. Two principal forms of the molecule are readily distinguishable on the basis of their absorption spectra (Fig. 14.1)
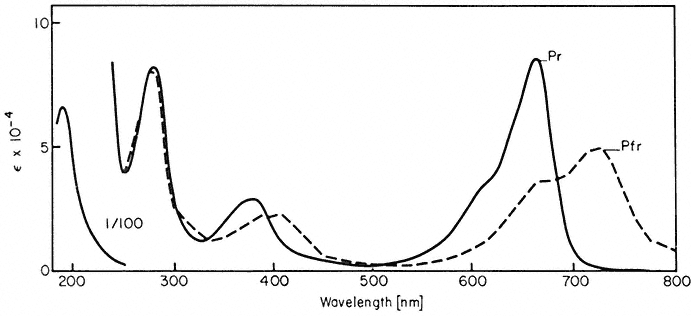
Figure 14.1
Absorption spectra of purified oat phytochrome following saturating
irradiations with red and far-red light (after Anderson et al., 1970).
and biological activity. The Pr form (= red-absorbing, lMax = 660 nm) is considered biologically inactive, whereas the Pfr form ( = far-red-absorbing, lMax = 730 nm) is considered to be the active species i.e. capable of inducing a biological response.
The two forms are reversibly interconvertible by red and far-red light ('phototransformation'). In addition, whereas the Pr form is stable in the dark, Pfr can revert thermally to Pr ('dark reversion') or undergo an irreversible loss of photoactivity ('destruction'). These properties are schematically represented thus:
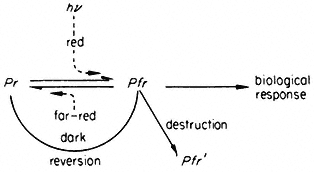
Red light absorbed by Pr converts the molecule to the Pfr form which in turn induces a measurable biological response. Far-red light, dark reversion and destruction provide alternate pathways for the removal of Pfr and thereby the potential for reversing induced responses. These properties endow the molecule with the capacity to function as a reversible biological switch, monitoring the environment for the presence, absence, intensity and spectral quality of photomorphogenically active light.
In molecular terms, phytochrome is considered to mediate the transmission of the light stimulus to the' cell via photoconversion to an active effector. Two major theories on how the cellular response system recognizes the effector have been advanced. One proposes phytochrome interaction with the genome; the other, interaction with cellular membranes.
14.3—
The Phytochrome Molecule
14.3.1—
Molecular Properties
The purified phytochrome molecule is a water-soluble chromoprotein containing less than 4% carbohydrate (Briggs & Rice, 1972). The native monomer is a polypeptide of 120,000 daltons but can form higher molecular weight aggregates. The chromophore is thought to be a linear tetrapyrrole with some evidence suggesting that there is one chromophore per monomer (Tobin & Briggs, 1973). The proposed chromophore structures, their postulated linkages to the protein and a possible photo-isomerization mechanism are shown in Fig. 14.2 (Rüdiger, 1972).
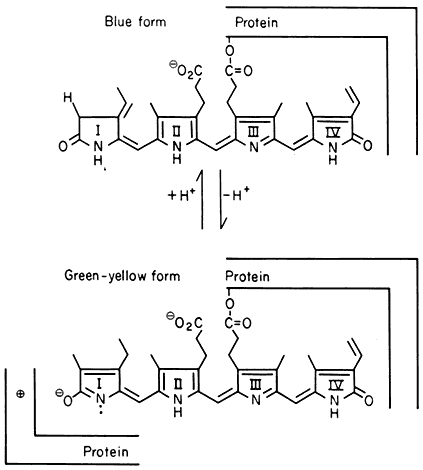
Figure 14.2
Proposed structure for the phytochrome chromophore, its linkage to the
protein and possible phototransformation mechanism (after Rüdiger, 1972).
The 'blue' form is thought to correspond to P r and the 'green-yellow' form to Pfr.
Information on differences in the molecular organization of the Pr and Pfr species has been sought in the hope that this might provide some insight into potential reaction mechanisms. Differences in the chromophore environment are evident from the absorption (Fig. 14.1), circular dichroism and optical rotatory dispersion spectra in the visible region (Kroes, 1970). Changes in protein conformation during photoconversion are also implied from low temperature and freeze-dry studies (Spruit & Kendrick, 1973; Kendrick, 1974). However, the post-conversion differences in the Pr and Pfr protein configurations are apparently only quite small as revealed by a variety of spectral and chemical methods (Briggs & Rice, 1972). Differences in surface residues are suggested by the ultraviolet difference spectrum (Tobin & Briggs, 1973); the differential reactivity of Pr and Pfr toward glutaraldehyde (Roux, 1972), and N -ethy maleimide (Gardner et al., 1974); and the differential electrostatic binding of Pr and Pfr to ribosomal material in plant extracts (Quail, 1975b).
14.3.2—
Photoconversion Reactions
Kinetic analysis following flash excitation of phytochrome indicates that the forward reaction (Pr ®Pfr ) requires several seconds to complete, whereas the reverse transformation (Pfr ®Pr ) is apparently complete by 20 to 30 msec
(Linschitz et al., 1966; Linschitz & Kasche, 1967). Several intermediates on separate pathways for the forward and reverse reactions have been characterized spectroscopically (Kendrick & Spruit, 1973). The first photochemical intermediate on both pathways appears to result from isomerization of the chromophore only with no change in protein structure. Subsequent dark relaxations apparently involve conformational changes in the protein moeity as well as further chromophore re-arrangements. The actual mechanism involved in chromophore photo-isomerization is uncertain, although tautomerization of the pyrrole group (Fig. 14.2) plus a cross-exhange of protons between chromophore and protein is a currently favoured hypothesis (Lhoste, 1972).
The phototransformation of a static population of phytochrome molecules can be described by the expression (Butler, 1972):

where l = wavelength; Il = intensity; t = duration of irradiation; Erl and Efrl = the extinction coefficients at l for Pr and Pfr respectively; fr and ffr = the quantum yields for Pr and Pfr respectively. At t =¥ a photo-equalibrium will be established and the pigment will oscillate ('cyc'e') between the two forms at a rate which is a function of the total absorption of the two species. The ratio of Pfr to Pr will remain constant. In the absence of any net loss or gain of phytochrome molecules in the population (a 'closed' system), this ratio will be wavelength dependent but irradiance independent according to the formula:

In the living cell, however, synthesis and destruction of phytochrome (see 14.3.3.2 below) must be taken into account. This transforms the system into an 'open' one where net loss or gain of pigment molecules can and do occur. For short term irradiations (about 5 minutes), where photo-equilibrium is rapidly established, no significant change in total phytochrome (Ptot ) occurs and Eq. 14.2 is a good first approximation. Under these conditions the [Pfr ]:[Pr ] ratio in the cell will be irradiance independent but wavelength dependent. Under long term continuous irradiations, however, synthesis and destruction become significant parameters with the result that the ratio [Pfr ]:[Pr ] becomes irradiance, as well as wavelength, dependent (Schäfer & Mohr, 1974; Schäfer, 1975).
Experimentally the kinetics of phototransformation have been shown to be first order both in vivo (Schmidt et al., 1973) and in vitro (Butler, 1961). Likewise, both the rates of photoconversion (Fig. 14.3) and the short term photosteady state ratio of Pr to Pfr (Fig. 14.4) have been demonstrated to be wavelength dependent in a manner consistent with the measured absorption spectra (Butler et al., 1964; Hanke et al., 1969).
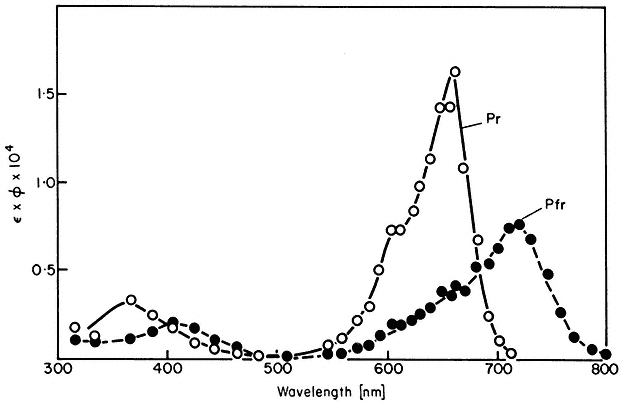
Figure 14.3
Action spectra of photochemical transformations of P r and Pfr in
solution. The extinction coefficient Î is in litre mol1 cm–1 and the
quantum yield f is in mol Einstein–1 (after Butler et al., 1964).
Note that the rate and extent of photoconversion are dependent on the wavelength, irradiance and time of irradiation below photo-equilibrium (Eq. 14.1). The product of time and irradiance determines the total number of quanta or the light 'dose' administered to the system. The wavelength determines how
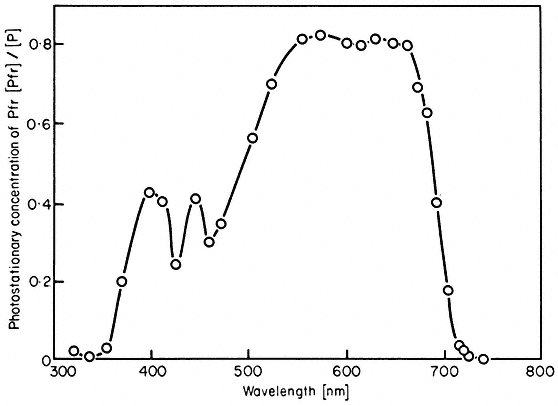
Figure 14.4
Proportion of phytochrome in the Pfr form at photoequilibrium
in vivo (Sinapis hooks) as a function of wavelength
(after K.M. Hartmann and C.J.P. Spruit in Hanke et al., 1969).
efficiently the incident quanta are absorbed by the pigment (Fig. 14.1). Note also that total photoconversion of Pr to Pfr is not possible (Fig. 14.4). The maximum attainable is about 80% Pfr in the red region of the spectrum. This is because there is no wavelength where Pr absorbs and Pfr does not (Fig. 14.1). Conversely, more than 97% of the phytochrome can be converted to the Pr form by wavelengths longer than 737 nm (Hartmann & Cohnen Unser, 1973) as the Pfr absorbance exceeds that of Pr in that region of the spectrum. The photosteady state ratio of Pfr/Pr can be conveniently manipulated with monochromatic light, particularly between 660 and 750 nm (Fig. 14.4). This procedure has been used to advantage in several physiological experiments.
For many years the only quantitative assay for phytochrome has been the spectrophotometric measurement of its photoreversible absorbance changes (Fig. 14.1) (Butler et al., 1959; Spruit, 1972). This procedure can measure phytochrome both in vivo and in vitro. However, it is unsuitable for use with green tissue and provides no index of the integrity of large regions of the protein moeity. The recent successful immunocytochemical detection of phytochrome now provides a second assay for the pigment (Coleman & Pratt, 1974).
14.3.3—
Dark Reactions
'Dark reversion' and 'destruction' are the so-called dark reactions of phytochrome. Neither the molecular bases nor the physiological significance of these processes is well understood.
14.3.3.1—
Dark Reversion
Pr is thermodynamically stable and can only be converted to Pfr by light (Lhoste, 1972). Pfr, in contrast, is metastable and can therefore revert thermally to Pr in the dark.
In vivo, dark reversion occurs in most dicotyledons but not monocotyledons, whereas phytochrome from both sources reverts in vitro (Frankland, 1972; Briggs & Rice, 1972). The process in vivo appears to be first order and rapid. In several plants reversion is complete within 30 minutes at 20ºC although only 15% to 20% of the Pfr molecules are involved. The remainder continue to undergo 'destruction' for a considerable period after reversion has ceased. Separate 'reversion' and 'destruction' pools of Pfr have been postulated to account for this apparent anomaly (Schäfer & Schmidt, 1974). Discontinuities in Arrhenius plots of the extent of reversion suggest that the process might be membrane associated (Schäfer & Schmidt, 1974).
14.3.3.2—
Synthesis and Destruction
Dry seeds contain phytochrome (Spruit & Mancinelli, 1969). Rapid, early increases in the photometrically detectable pigment (Ptot ) during imbibition are apparently due to rehydration of the molecule rather than synthesis (Tobin et al.,
1973). The pigment can be stored as either Pr or Pfr and rehydrated in the stored form (Vidaver & Hsiao, 1972). This suggests an explanation for the appearance of Pfr in the dark, sometimes observed in seeds (Rollin, 1972). Further increases in Ptot during the growth of etiolated seedlings result from de novo synthesis of new molecules in the Pr form (Quail et al., 1973b). The pigment accumulates to high levels in the dark ultimately reaching a plateau (Fig. 14.5).
'Destruction' is the disappearance of photometrically detectable Pfr without the concomitant appearance of equimolar quantities of Pr (Frankland, 1972). This decrease in photoactivity is paralleled by a loss of immunologically detectable phytochrome (Coleman & Pratt, 1974). Since no recycling of the protein moeity occurs, 'destruction' would appear to be a genuine degradative process (Quail et al., 1973b). Some evidence suggests that this process may be enzymatic (Kidd & Pratt, 1973). Destruction is temperature dependent, but the absence of discontinuities in Arrhenius plots suggests that in contrast to dark reversion, it is not membrane associated (Schäfer & Schmidt, 1974). Destruction is observed in both monocotyledons and dicotyledons with half-times ranging from 20 minutes to 4 hours (Frankland, 1972; Schäfer et al., 1973; Kidd & Pratt, 1973).
Destruction of Pfr occurs both in the dark following brief irradiations and in continuous light. In the dark, destruction rapidly removes all unreverted Pfr leading to a short-term decline in Ptot. The reduced pigment levels are replenished, however, as the dark period proceeds by de novo synthesis of new Pr molecules (Quail et al., 1973b). In continuous light, initially high Ptot levels decline at a rate proportional to the photosteady-state Pfr concentration (Frankland, 1972) ultimately reaching a new plateau (Fig. 14.5). This new
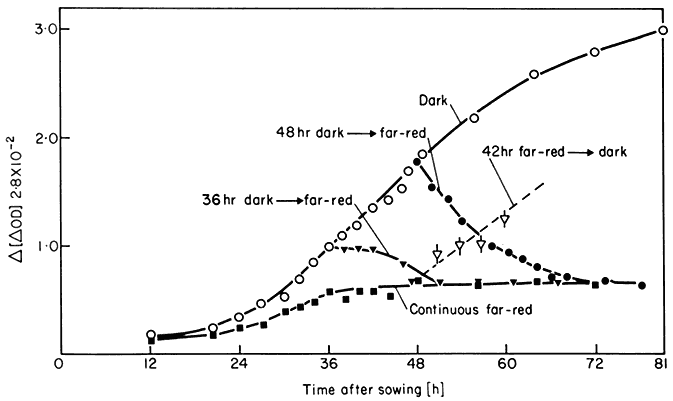
Figure 14.5
Total phytochrome levels in Sinapis cotyledons as function of time in the dark
(o); continuous far-red (


48 h dark ® far-red (

plateau represents a steady-state equilibrium between synthesis and degradation (Schäfer et al., 1972; Quail et al., 1973b). Pr synthesis appears to be a continuous zero order process, itself unaffected by light. The Ptot level is then regulated against this background by the disparate first order degradation rate constants for Pr and Pfr.
The plateau level of Ptot established at the steady-state by prolonged irradiations is a function of both wavelength and irradiance (Schäfer & Mohr, 1974; Schäfer, 1975). The Pfr level, in direct contrast however, is independent of both irradiance and wavelength under these conditions. This has the extremely important consequence that, whereas the ratio of [Pfr ]:[Ptot ] will change depending on wavelength and irradiance, the absolute level of Pfr will be the same under all continuous irradiation conditions once the steady-state has been established. This has important implications for the interpretation of the effects of prolonged irradiations.
14.3.3.3—
Physiological Significance of Dark Reactions
The dark reactions provide a mechanism for the light independent removal of Pfr and thereby the potential for a dark period to reverse light-induced responses which require the sustained presence of the effector. Furthermore, as the disappearance of Pfr is a time dependent process the system has the potential for measuring time. In principle, therefore, phytochrome should enable the plant to distinguish between light and dark and to time the dark period. Initially it was thought that this so-called 'hour-glass' principle was the basis for phytochrome-controlled photoperiodism (see Vince, 1972). Supporting evidence is scant, however, and this theory has now fallen into disrepute. Some systems do, nevertheless, respond to the timed disappearance of Pfr in the dark. An example is accumulation of the enzyme lipoxygenase (Oelse-Karrow & Mohr, 1973).
During prolonged irradiations, synthesis and destruction appear to have the additional role of maintaining a constant absolute level of Pfr irrespective of wavelength (Schäfer, 1975). This implies that Pfr per se is not the effector of high irradiance responses (see 14.4.1.2).
14.3.4—
Localization
The distribution of phytochrome is highly specific at the tissue and cellular levels as determined photometrically (Briggs & Siegelman, 1965) and immunocytochemically (Pratt & Coleman, 1971; 1974). In dicotyledons, the highest pigment levels are in the apical regions. In etiolated grass shoots, large quantities occur in parenchyma cells near the tip of the coleoptile and in the rapidly differentiating tissue near the shoot apex. High levels of the pigment are also found in root cap cells.
Attempts to establish the subcellular localization of phytochrome fall into two categories: (a) measurement of phytochrome-induced responses having a
spatial or vectorial component from which the photoreceptor location can be inferred; (b) direct measurements of the pigment itself, either in situ or in subcellular fractions.
The pattern of chloroplast movement in the alga Mougeotia in response to polarized red and far-red microbeams has been taken as evidence that phytochrome located and orienled on or near the plasmalemma controls this response (Haupt, 1972b). The directional growth of the germ tubes of Dryopteris in polarized light has been interpreted similarly (Etzold, 1965). The change in ion flux associated with phytochrome mediated leaflet movement (Satter & Galston, 1973), root tip adhesion to glass surfaces (Tanada, 1968), and changes in bioelectric potentials (Newman & Briggs, 1972) are also indicative of phytochromecontrolled changes in surface properties but not necessarily that the pigment is a permanent membrane component. A report of phytochrome-controlled development of isolated etioplasts in vitro (Wellburn & Wellburn, 1973) implies the presence of functionally active pigment in or on the organelles. More recently a rapid, phytochrome-mediated change in the level of gibberellin extractable from an etioplast-rich fraction in response to in vitro irradiations has been demonstrated (Evans, 1975; Evans & Smith, 1976a; Cooke & Saunders, 1975). A red/far-red reversible reduction of NADP in vitro in response to irradiation of a mitochondria-rich fraction has also been reported (Manabe & Furuya, 1974). Furthermore, phytochrome has been detected spectrophotometrically in both etioplast- (Evans & Smith, 1975) and mitochondria-rich fractions (Manabe & Furuya, 1974).
Both spectrophotometric and immunological techniques have been used for direct, in situ measurements of the intracellular distribution of phytochrome. The presence of the pigment in the nucleus has been claimed on the basis of microspectrophotometric scans of cells (Galston, 1968) but these data have been challenged (Kendrick & Spruit, 1972; Tobin et al., 1973). The cytochemical visualization of phytochrome antibody in non-irradiated tissue sections indicates a general distribution of the photoreceptor throughout the cytoplasm in addition to an association with nuclei and plastids (Pratt & Coleman, 1971). Brief red irradiation prior to fixation causes the pigment in some tissues to concentrate in discrete, as yet unidentified regions of the cytoplasm (Mackenzie et al., 1974). Non-saturating irradiations of maize coleoptile segments with red and far-red light polarized normal to the longitudinal axis were found to photoconvert about 20% more phytochrome than when polarized parallel to this axis (Marmé & Schäfer, 1972). This was interpreted as indicating that phytochrome is located and oriented in the plasmalemma. In considering the locational and orientational rigidity of phytochrome implied from polarized light studies the known rapid and highly fluid lateral and rotational diffusion of other membrane proteins should be borne in mind (Cone, 1972; Singer, 1974).
Cell fractionation procedures have also been used in attempts to localize phytochrome in subcellular components. The well-known precipitation of the pigment protein at low pHs (

has been overlooked in some studies leading to claims of associations of phytochrome with mitochondria (Gordon, 1961) and plasmalemma (Marmé et al., 1971). Little of the pigment (< 10%) sediments from homogenates of nonirradiated tissue at neutral pH at forces up to 144,000 × g (Rubinstein et al., 1969; Siegelman & Butler, 1965). Red irradiation prior to extraction, however, substantially enhances the level of phytochrome subsequently associated with pelletable material (Quail et al., 1973a). Irradiation of extracts from dark grown material has a similar effect (Marmé et al., 1973). Initially claims were made of the isolation of a phytochrome-containing membrane fraction that could be reversibly 'solubilized' by withdrawal of Mg2+ (Marmé et al., 1973; 1974). More recently, however, the pigment in this fraction has been shown to be associated with degraded ribonucleoprotein (RNP) material, probably of ribosomal origin (Quail, 1975b). This association apparently results from the preferential electrostatic adsorption of Pfr onto ribosomal material—either free or membrane-bound in the endoplasmic reticulum (Williamson et al., 1975). Whether such an association is artefactual or biologically meaningful is yet to be established. A recent promising variation on this approach is the use of glutaraldehyde in an attempt to immobilize the pigment in the cell prior to extraction (Yu, 1975.)
The existence of meaningful phytochrome-membrane interactions are by no means excluded by the above findings. It has been shown, for example, that phytochrome can mediate photoreversible conductance changes in artificial lipid membranes (Roux & Yguerabide, 1973). The suggestion that phytochrome might function as a stereospecific protein ligand capable of interaction with cellular membranes has been made (Quail & Schäfer, 1974; Boisard et al., 1974).
14.4—
Phytochrome Physiology
The biological responses attributed to phytochrome can be usefully characterized in terms of three different but interrelated sets of criteria: (a) the nature of the involvement of light in the inductive process; (b) the temporal expression of the induced response; and (c) the type of cellular or developmental process affected.
14.4.1—
Induction-Reversion and High Irradiance Responses
Two types of light-controlled phenomena have been attributed to phytochrome—the so-called 'induction-reversion' and 'high irradiance' (HIR) responses (Mohr, 1972). This terminology arises from the irradiation conditions under which the responses are observed and suggests a fundamental difference in the manner in which the phytochrome molecule transmits the light signal to the cell in each case. It does not necessarily reflect an intrinsic property of the actual, biological parameter being monitored. Some parameters display both modes of response, others only one.
14.4.1.1—
Induction-Reversion Responses
These are the classical phytochrome responses (Borthwick, 1972). A change in the biological parameter being monitored is induced by a brief irradiation of low intensity red light and reversed by a subsequent far-red pulse. The accumulation of anthocyanin in Sinapis illustrates this point (Fig. 14.6). Another well known example is lettuce seed germination. This is repeatedly photoreversible for up to 100 alternate red and far-red irradiations (Borthwick, 1972).
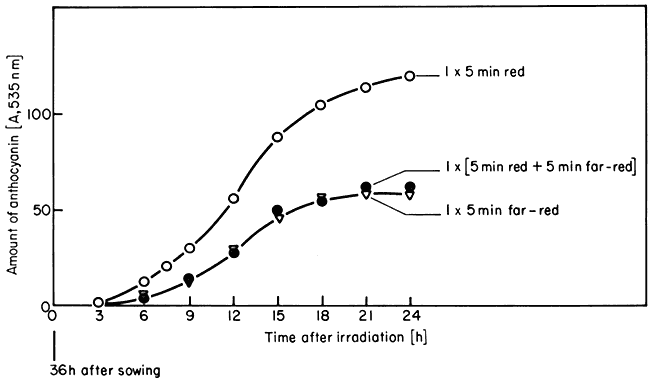
Figure 14.6
Accumulation of anthocyanin in Sinapis in the dark following irradiation
treatments at time zero with 5 min red (o), 5 min far-red (Ñ ), or 5 min
red followed immediately by 5 min far-red (

Mohr et al., 1971).
This simple red/far-red photoreversibility forms the basis of the concept that Pfr is the biologically active form of the pigment whereas Pr is inactive. Attempts to quantify the relationship between the number of Pfr molecules formed and the magnitude of the induced response have been both indirect and direct.
Indirect correlations are based on the premise that observed increases in the magnitude of the response with increasing light dose are a function of the degree of photoconversion of Pr to Pfr, i.e. the more quanta, the more Pr is converted to Pfr and therefore the greater is the response. The increase in anthocyanin in response to increasing doses of red light (Table 14.1) illustrates this point (Lange et al., 1971). The so-called law of reciprocity (irradiance × time = constant) must hold for the light doses used for this interpretation to be valid (see 14.3.2). This establishes that the magnitude of the response is directly proportional to the total number of incident quanta regardless of the time or irradiance of the
irradiation providing those quanta (Table 14.1). The effects of irradiance level during the brief irradiations used in induction-reversion experiments are thus attributed entirely to the degree of photoconversion.
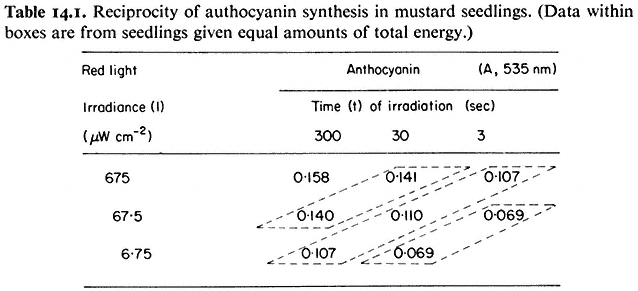
Biological action spectra are an extension of this principle (Fig. 14.7). The magnitude of the response at different wave-lengths is interpreted to be a function of the relative effectiveness of the quanta at those wavelengths in the phytochrome photoconversion process. The close agreement between the action spectra of several biological responses on the one hand (Fig. 14.7) and those of the phototransformation reactions of the isolated pigment on the other (Fig. 14.3) lends strong support to this notion (Borthwick, 1972; Shropshire, 1972). In these cases a seemingly good correlation exists between Pfr level and response magnitude.
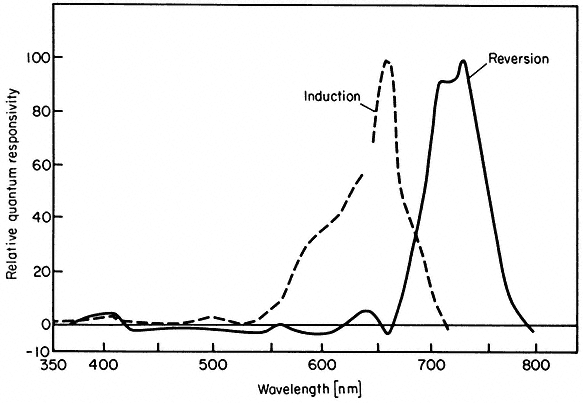
Figure 14.7
Action spectra for induction and reversion of plumular hook opening in bean seedlings
(after Withrow et al., 1957).
In contrast, however, the majority of rigorous attempts to demonstrate a direct quantitative correlation between the photometrically detectable Pfr level and the relevant biological response in the same system have been unsuccessful (Hillman, 1972). The reasons for this are not understood. An apparent exception is lipoxygenase levels in Sinapis (Oelze-Karrow & Mohr, 1973).
Increases in response with increasing doses of quanta must eventually saturate. If the level of Pfr is rate-limiting the photoresponse will saturate when the photoconversion process is saturated i.e. when photoequilibrium is reached. If the response system itself is rate-limiting the response may saturate well before photoequilibrium. Examples of both extremes have been observed (Hillman, 1967). Light doses which saturate the photoconversion of Pr to Pfr do not appear to saturate the inhibition of mesocotyl lengthening in Avena (Loecher, 1966), nor the accumulation of anthocyanin in Sinapis (Drumm & Mohr, 1974). In contrast, inhibition of lipoxygenase accumulation is saturated by very low (< 3%) Pfr levels (Mohr, 1972). Other photoresponses fall between these extremes with many being saturated at less than 80% Pfr (Hillman, 1967). In addition, whereas many parameters, such as anthocyanin formation, show a graded response, others, such as lipoxygenase accumulation, respond in an all-or-none fashion to changes in Pfr level, suggesting some form of cooperative, threshold mechanism (Oelze-Karrow & Mohr, 1973).
Implicit in the far-red reversibility of an induced response is that Pfr can act in the dark. Light is strictly a trigger. The magnitude and multiplicity of the responses indicate an extensive amplification mechanism. Unlike photosynthesis where light energy is converted stoichiometrically with quantum yields of less than 1.0, the low irradiances which actuate these phytochrome responses lead to final quantum yields well in excess of unity (Galston, 1974).
14.4.1.2—
High Irradiance Responses (HIR)
If Pfr is, as postulated, the active, effector, it is clear that tripartite correlations between light dose, Pfr level and response magnitude will only be expected for irradiations terminated prior to photoequilibrium. Once photoequilibrium has been established, Pfr le vels are no longer irradiance dependent whether for short term (Eq. 14.2) or long term (Schäfer, 1975) irradiations. No further increase in the response should result therefore from further increases in irradiance after photoequilibrium.
Some parameters do, however, show a strong irradiance dependence at photoequilibrium. This effect is termed the high irradiance response (HIR) (Mohr, 1969). Such responses are observed with continuous irradiation where a phytochrome photosteady-state is rapidly established and maintained for prolonged periods. The accumulation of anthocyanin in continuous far-red light illustrates this point (Fig. 14.8) (Lange et al., 1971). The rate of accumulation is a function of the irradiance. This effect is only maintained, however, as long as the irradiation continues. The irradiance-enhanced response rate reverts rapidly
to that of the dark controls when irradiation ceases, and resumes again upon further irradiation (Fig. 14.9). Reciprocity is not demonstrable for the HIR and, in some cases e.g. lettuce hypocotyl lengthening, the variable itself does not exhibit the classical red/far-red reversible response (Hartmann, 1966). Anthocyanin accumulation, in contrast exhibits both modes of response (Figs. 14.6 and 14.8).
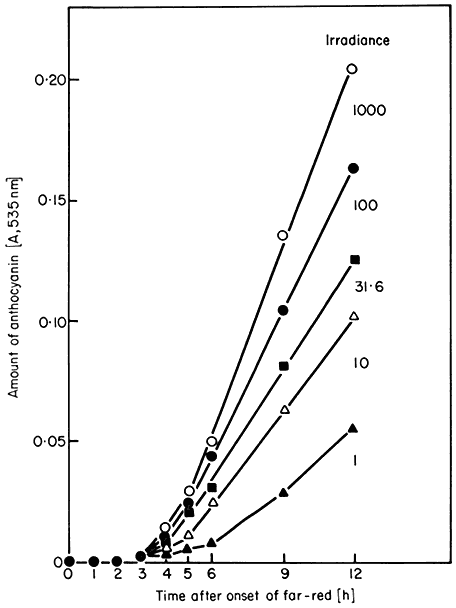
Figure 14.8
Accumulation of anthocyanin in Sinapis under high irradiance
conditions. Seedlings were held in continuous far-red light of
varying irradiance. All irradiances are expressed relative
to the arbitrary value of 1,000 (= 350 µW. cm–2 )
(after Lange et al., 1971).
Action spectra of HIR have peaks in the blue and far-red (Borthwick et al., 1969; Mohr, 1969). The most extensively studied response is that of the inhibition of lettuce hypocotyl lengthening (Hartmann, 1967; see also Fig. 14.10). The seedlings were irradiated continuously for 18 hours with monochromatic light of different wavelengths and irradiances. As with other HIR there is no coincidence of the observed spectrum with the absorption maxima of either Pr or Pfr.
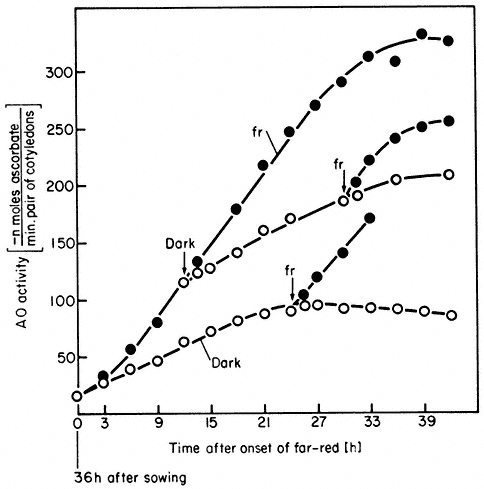
Figure 14.9
Accumulation of ascorbate oxidase activity in Sinapis under
high irradiance conditions. Seedlings were either retained in
the dark (o) or continuous far-red

seedlings from far-red to the dark or vice versa at
various times is indicated by the arrows (̄ )
(after Drumm et al., 1972).
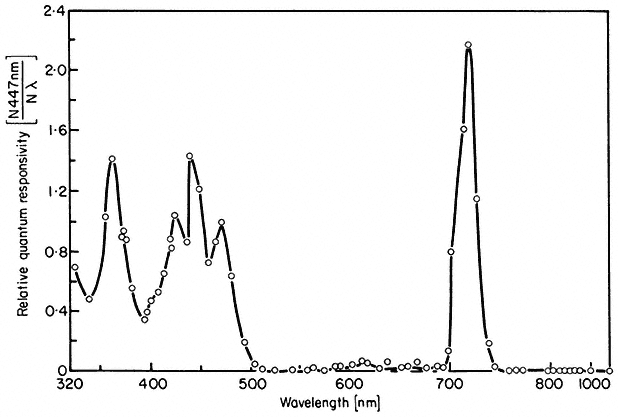
Figure 14.10
Action spectrum for inhibition of lettuce hypocotyl
lengthening under continuous irradiation
(after Hartmann, 1967).
The conclusion that phytochrome mediates the high irradiance effects derives from other data using the same plant system (Hartmann, 1966). Prolonged irradiations with two wavelengths which were relatively ineffective when given separately (658 nm and 766 nm) were highly effective when given simultaneously. The maximum effect with these and other wavelength pairs always occurred where the photosteady-state Pfr concentration was about 3%. This agrees well with the peak of activity observed at 720 nm with single wavelength monochromatic light (Fig. 14.10) also known to establish about 3% Pfr at photoequilibrium (Fig. 14.4). Furthermore, the effectiveness of a single wavelength irradiation at 717 nm could be nullified by simultaneous irradiations of either 658 nm or 759 nm. These wavelengths would shift the photoequilibrium away from 3% Pfr towards higher or lower values respectively.
The question remains, however, as to how both the irradiance and wavelength dependency of the HIR can be explained in terms of phytochrome. The response is unlikely to be a function of the absolute Pfr level as this is irradiance and wavelength independent with prolonged irradiations (Schäfer, 1975). The rate at which the pigment molecule oscillates between the two forms at photoequilibrium is, on the other hand, strongly irradiance and wavelength dependent. Accordingly, the HIR has been rationalized to be some function of the cycling rate of phytochrome. The effector molecule has been postulated to be some 'excited form of Pfr', denoted Pfr* (Schopfer & Mohr, 1972). No direct evidence for such a species is available, however, and its purported action has been questioned on the basis of dual wavelength experiments (Hartmann & Cohnen Unser, 1973). High levels of phytochrome intermediates are maintained at high irradiances (Kendrick & Spruit, 1973a) but the possibility that these are HIR effectors is unlikely because of the nature of the action spectra (Hartmann, 1966).
14.4.1.3—
A Unitary Model
Schäfer (1975) has recently developed a single, formalistic model which can theoretically account for both induction-reversion and HIR responses, whether graded or cooperative, in terms of phytochrome. The pigment is postulated to be a bimodal ligand with the forms Pr and Pfr which interact with receptor sites also having dual forms, X and X':
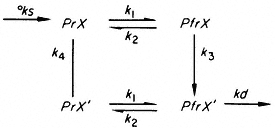
where °ks and kd are the rate constants for synthesis and destruction of phytochrome respectively; k1 and k2 rate constants for the photoconversion reactions; and k3 and k4 rate constants for the X®X' and X'®X transitions.
The irradiance and wavelength dependencies of the HIR response then become explicable in terms of the flux rates through the cycle under continuous irradiation. The basic conclusions reached are that PfrX' is the effector element in induction-reversion responses, whereas PfrX or the flux k3 . PfrX is the effector element in HIR. The molecular nature of X and the mechanism of action of the effector elements are, however, unresolved questions.
14.4.1.4—
Modes of Light Signal Transmission
Given that phytochrome mediates both induction-reversion and HIR, it is clear that the photoreceptor utilizes the incoming light signal differently in each case. Whereas induction-reversion responses are induced by a red pulse and can develop in the subsequent dark period, HIR require a continuous light energy input to sustain the response. Increases in irradiance lead to increases in response magnitude in both cases but the effect is interpreted differently for each. For induction-reversion responses the irradiance effect is only observed prior to photoequilibrium; is interchangeable with time of irradiation (reciprocity holds); and is interpreted as reflecting the effectiveness of the total light dose in determining the degree of photoconversion of Pr to Pfr. Pfr is considered the effector molecule and some form of Pfr -response stoichiometry is expected. For HIR, on the other hand, the irradiance effect is observed at photoequilibrium; is not interchangeable with time of irradiation (reciprocity does not hold); and is interpreted as being some function of the phytochrome cycling rate. The precise effector molecule or process is uncertain. For induction-reversion responses light is viewed simply as a trigger and as having no further direct role in the inductive function of Pfr. The light signal is 'stored' in the Pfr form for subsequent utilization. HIR, in contrast, require a sustained, direct interaction of the photoreceptor with the incident excitation energy. This indicates that these effects are light-driven as distinct from being light-triggered. Light appears to have a direct role in the inductive function of the pigment, the energy input being rapidly dissipated.
The suggestion that photosynthesis or cyclic photophosphorylation might in some way be responsible for HIR has been advanced but several pieces of evidence argue against this (Mohr, 1972). Speculative suggestions that phytochrome might function as a photocoupler (Quail, 1975a) or a specific, lightdriven permease (Smith, 1970) in the HIR have also been advanced but not substantiated.
14.4.2—
Response Kinetics
Phytochrome is considered to trigger, in some way, a chain of events leading sooner or later to a measurable biological response. The initial triggering of those processes necessary for the development of the response can be termed phytochrome 'action'; and the appearance of a measurable change in the
parameter being monitored can be called response 'expression'. This leads to the recognition of three categories of phenomena: (a) rapid action/rapid expression; (b) rapid action/delayed expression; and (c) delayed action/delayed expression responses. 'Rapid' here arbitrarily means

14.4.2.1—
Rapid Action/Rapid Expression Responses
As phytochrome action must either coincide with or precede expression, rapid action can be implied from the kinetics of the expression alone in these cases. A red/far-red reversible change in electric potential in Avena coleoptiles within 15 seconds of the start of irradiation is the most rapid phytochrome-mediated phenomenon thus far reported (Newman & Briggs, 1972). Similar changes have been observed in the biolelectric potential of mung bean root tips exposed to successive red and far-red irradiations (Jaffe, 1968; Racusen & Miller, 1972). Such changes had earlier been inferred from the red/far-red reversible adhesion of root tips to negatively charged glass surfaces (Tanada, 1968). Both responses are detectable within 30 seconds of the start of irradiation (Fig. 14.11). These changes in surface charge are interpreted to indicate changes in the plasmalemma. Fluorescent probe studies support this notion (Racusen, 1973). Red light also induces H+ efflux from root tips (Yunghans & Jaffe, 1972).
Phytochrome regulates leaflet movement in Mimosa, Albizia and Samanea (Satter & Galston, 1975). This movement is accompanied by an energy-dependent transfer of K+ ions between the ventral and dorsal motor cells of the pulvinus. Both effects are detectable 10 minutes after red or far-red irradiations. The changes in K+ are correlated with changes in transmembrane potential (Racusen & Satter, 1974). Furthermore, red/far-red regulated changes in surface charge similar to those of root tips are detectable 30 to 120 seconds after irradiation. These effects are also strongly indicative of phytochrome-mediated changes in plasmamembrane properties.
Phytochrome-regulated changes in the rate of plasmolysis have been detected in Mougeotia within 6 minutes of the onset of red irradiations (Wiesenseel & Smeibidl, 1973). A change in plastid orientation is also evident in this alga in less than 10 minutes after a red pulse (Haupt, 1972a). The latter effect can only be fully reversed by far-red during the first minute after red light. Thus potentiation of the response has begun within 1 minute of photoconversion. Available data indicate that the effective phytochrome is located on or near the plasmalemma. Contractile fibrils appear to be responsible for the chloroplast movement (Schönbohm, 1973).
Red light (15 seconds) induces an increase in growth rate in coleoptile tips within 60 seconds of the start of irradiation (Weintraub & Lawson, 1972). The effect is partially reversed if far-red immediately follows the red. Inhibitors of transcription and translation are without effect. As the cell must regulate wall extension through the plasmalemma, this response might also represent a
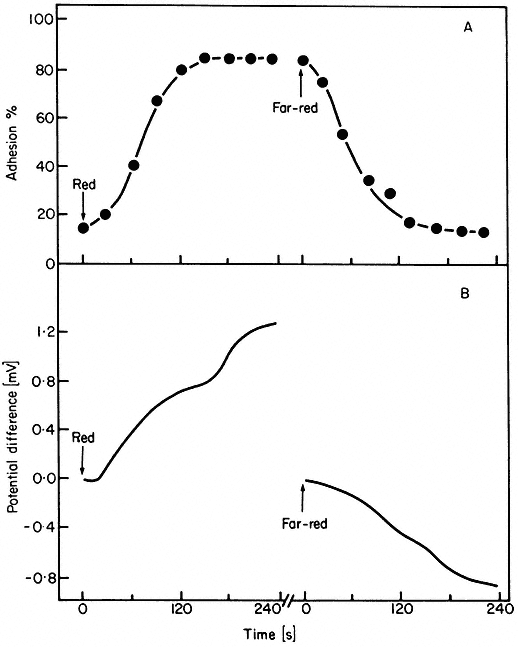
Figure 14.11
Kinetics of (A) root tip adhesion to a negatively charged glass
surface and (B) the development of a biolectric potential across
the root tip in response to irradiation with red or far-red light
(after Jaffe, 1968).
phytochrome-mediated membrane change. Rapid changes in ATP levels, induced by red and, in some cases, partially reversed by far-red, have been reported but no consistent pattern is obvious (Sandmeier & Ivart, 1972; Yunghans & Jaffe, 1972; White & Pike, 1974).
Rapid transitory increases in hormore levels in response to red light have been known for some time (Reid et al., 1968; Beevers et al., 1970; van Staden & Wareing, 1972). Unequivocal evidence of far-red reversibility has generally been lacking in the past, however, often because of poor experimental design. Recently, the level of gibberellin activity extractable from etioplast-rich preparations from grass leaves has been shown to increase 3-fold within 5 minutes of the termination of a 5 minute red irradiation of the isolated fraction (Evans, 1975; Evans & Smith, 1976a). This effect is reversed by far-red light given immediately after the red. It is postulated that phytochrome causes the movement of gibberellin across the etioplast envelope into the ambient medium.
These observations, and those made with mitochondria-rich fractions (Manabe & Furuya, 1974), are rapid, of potential physiological significance, and suggestive of in vitro phytochrome-mediated changes in the functional properties of membranes in the isolated fractions.
Lipoxygenase accumulation in Sinapis responds to changes in Pfr level in less than 5 minutes under appropriate conditions (Oelze-Karrow & Mohr, 1973). It has been concluded that the level of the enzyme in the cotyledons is controlled by phytochrome in the hypocotyl hook, through a highly cooperative threshold mechanism that responds to, and is saturated by, Pfr levels of 1–2% of the total pigment (Oelze-Karrow & Mohr, 1974). Other rapid, inter-organ transfer of phytochrome signals have also been reported (de Greef & Caubergs, 1973). Some form of biophysical transmission system has been postulated, with the membrane continuum of the plasmodesmata as a suggested candidate (Oelze-Karrow & Mohr, 1973).
14.4.2.2—
Rapid Action/Delayed Expression Responses
Rapid phytochrome action in these cases is deduced from the rate at which the response escapes susceptibility to photoreversal by far-red light following an inductive red pulse. The actual response may not be expressed for days after the irradiation although the inevitability of its appearance has long since been irreversibly established. Pfr is said to have 'potentiated' the response (Borthwick, 1972). The escape from reversibility is viewed as the Pfr -triggered reaction chain having progressed beyond those steps directly under phytochrome confrol.
The effect of a red light pulse on flowering in Pharbitis is only partially reversed by far-red given 30 seconds after the start of the red irradiation (Fredericq, 1964). After 3 minutes, far-red no longer reverses the effect. Thus although the flowering response itself is not expressed for several days, it is potentiated within seconds by Pfr. Flowering in Chenopodium album and Kalanchoe behaves similarly. A synergism between phytochrome and gibberellin in lettuce seed germination (Bewley et al., 1968) and the de-etiolation response of Pisum (Haupt, 1972a) are also in this category.
14.4.2.3—
Delayed Action/Delayed Expression Responses
These responses show a lag from irradiation to expression but are readily reversed by far-red over relatively long periods in the dark after the red pulse. Gradual escape from reversibility can occur but is relatively slow. This is viewed as indicating that the continued presence of Pfr is required over a relatively long period in the dark to maximise expression. An important feature of these responses is that dark reversion and destruction are continually and often rapidly depleting the Pfr pool during this period.
The vast majority of recorded red/far-red reversible responses, too numerous to catalogue, are included in this category (see Mitrakos & Shropshire, 1972;
Mohr, 1972; Smith, 1975). Lettuce seed irradiated for I minute with red light germinates up to 100% 24 hours later. Far-red reverses this effect up to 12 hours after the red irradiation but with decreasing effectiveness. Anthocyanin formation in Sinapis has a lag of 3 hours from irradiation to the onset of accumulation (Fig. 14.6). During this time the effect becomes decreasingly susceptible to reversal by far-red but escape is never complete. The in vitro protein synthetic capacity of 80s ribosomes from corn is enhanced by 5 minutes of red light prior to harvest (Travis et al., 1974). This effect is detectable within 30 minutes of red irradiation and escapes far-red reversibility within an hour.
14.4.3—
Response Manifestations
Phytochrome has a regulatory role in all major phases of plant growth and development. The changes in cellular biochemistry and physiology which underlie this regulation are detectable at almost any chosen level. Some responses are biophysical in nature (membrane potential), others biochemical (enzyme levels); some require protein synthesis (leaf expansion), others do not (leaf movements); some involve cell division (seed germination), others only cell expansion (plumular hook opening); some are restricted to the irradiated cells (leaf movements), others result from transmissable stimuli (floral induction); some are dependent on phytochrome-endogenous rhythm interactions (flowering), others appear independent (seed germination) (Galston, 1974; Satter & Galston, 1975).
When the nature of the responses is coupled with their kinetic properties a pattern emerges. In general, the most rapid responses are surface or membrane-associated phenomena, often physico-chemical in nature and independent of RNA and protein synthesis. Other cellular processes such as changes in enzyme levels mostly respond more slowly.
14.5—
Mechanism of Action
The molecular mechanism of phytochrome action is the central issue in phytochrome research. The term 'primary reaction of phytochrome' has been used to describe the first reaction in which the physiologically active species becomes involved (Mohr, 1972). Assuming Pfr to be the active species this reaction has been formalized:

where X satisfies the logical necessity of a reaction partner regardless of its nature. The 'primary action' of phytochrome then is presumably the induction of a functional change in the reaction partner (X ®X' ). These are useful formalistic treatments but say nothing of the actual molecular mechanisms involved.
Any molecular hypothesis of phytochrome action must provide an explanation for the specificity, multiplicity and amplification of the induced responses, and attempt to reconcile the seemingly disparate modes of light signal transmission in induction-reversion and high irradiance responses. Specificity here refers to the observed capacity of different plants, organs, adjacent cells in the same tissue and even different processes in the same cell to respond differently to the same effector molecule (Mohr, 1972). All major hypotheses hold the view that this property resides in the fundamental programming of the target cells themselves rather than in the phytochrome molecule.
Multiplicity refers to the vast diversity in form, direction, magnitude and timing of response expression per se (see 14.4.3). This can be accounted for by a suitable amplification mechanism. Two major hypotheses of phytochrome action, based on this rationale, have been advanced in recent years: the differential gene activation hypothesis (Mohr, 1966) and the membrane permeability hypothesis (Hendricks & Borthwick, 1967). The possibilities that plant hormones (Black & Vlitos, 1972) or acetylcholine (Jaffe, 1970) might be primary mediators of phytochrome effects have also been investigated but convincing supporting data are sparse (Tanada, 1972).
The gene activation hypothesis in its broadest sense is trivial, as gross morphogenetic changes such as flowering are unlikely to occur without some modification of gene expression. In its strictest sense the hypothesis implies that the primary action of phytochrome is to alter genetic activity. This requires direct Pfr -genome interaction. Data relevant to this problem come mainly from measurements of phytochrome-induced changes in enzyme activities and related phenomena (Mohr, 1972).
There are two major criticisms of this hypothesis. First, while the data so far obtained are consistent with the general framework of the concept, they provide no evidence that Pfr interacts directly with the genome. Increased incorporation of [-H]-uridine into high molecular weight RNA in continuous far-red light has been reported and interpreted as phytochrome-enhanced transcription of rRNA cistrons (Thien & Schopfer, 1975). Phytochrome-mediated de novo synthesis of enzyme proteins has also been demonstrated (Attridge, 1974; Acton & Schopfer, 1974) but this does not establish that control is at the gene level. Indeed, insensitivity of responses such as triose phosphate dehydrogenase (Cerff, 1974) and ascorbate (Schopfer, 1967) accumulation towards actinomycin D might suggest otherwise in these cases. Evidence of phytochrome-mediated activation of precursors of phenylalanine ammonia-lyase has been advanced (Attridge et al., 1974) but this point is controversial (Acton & Shopfer, 1975).
The second criticism is that a growing list of responses appear unlikely to be explained by changes in gene expression. These are in general the rapid action/rapid expression responses. These are more rapid than would be expected of gene regulation and are often insensitive to inhibitors of transcription and translation (Satter & Galston, 1973). Furthermore, lipoxygenase, the only enzyme responding rapidly enough to be placed in this category, is now postu-
lated to be controlled by phytochrome in a separate organ (Oelze-Karrow & Mohr, 1974).
The membrane hypothesis postulates that phytochrome interacts directly with cellular membranes i.e. the primary action of Pfr is to modify membrane properties. Indirect support for this notion derives from the fact that the majority of the most rapid responses are either surface phenomena or can be rationalized in terms of membrane changes (see 14.4.2.1). Direct evidence of an association of phytochrome with an identifiable cellular membrane is yet to be advanced however (see 14.3.4) although Evans and Smith (1976b) have recently reported the presence of phytochrome in fractions enriched for etioplast envelope membranes.
A postulated membrane locale of phytochrome action permits the formulation of a unitary hypothesis to account for all phytochrome-mediated phenomena. Both rapid and long term effects can be rationalized to have emanated from the same primary event. A single, fundamental alteration in membrane properties, perhaps simultaneously in several cellular membranes, provides an immediate mechanism for the generation and amplification of a multiplicity of secondary effects (Changeux, 1969). Such effects might include changes in ion flux, activation of membrane bound enzymes, altered compartmentalization and soon. Any or all of these might lead directly or indirectly to altered gene expression. Moreover, a phytochrome-membrane association suggests a possible basis for the dual function of the pigment in induction reversion and HIR responses. The opportunity would exist for the molecule to function either as a reversible, stereospecific membrane effector, via photoinduced changes in its protein conformation; and/or as a photocoupler (Quail, 1975a) or light driven permease (Smith, 1970) via pigment cycling.
The speculative nature of such suggestions, however, is evidence of our ignorance in this area. Indeed the basic concept of only a single, primary phytochrome reaction has been challenged (Mohr, 1974). It has been suggested instead that a multiplicity of reaction partners and corresponding primary reactions must be postulated to account for both graded and cooperative responses in the same system (Mohr et al., 1971). This would circumvent the second criticism of the gene regulation hypothesis and obviate any potential conflict between the two major hypotheses. The molecular mechanism of phytochrome action is clearly an open question.
Further Reading
Briggs W.R. & Rice H.V. (1972) Phytochrome: Chemical and physical properties and mechanism of action. Ann. Rev. Plant Physiol.23, 293–34.
Galston A.W. (1974) Plant photobiology in the last half-century. Plant Physiol.54, 427–36.
Hillman W.S. (1967) The physiology of phytochrome. Ann. Rev. Plant Physiol.18 , 301–24.
Mitrakos K. & Shropshire W. JR. (1972) Phytochrome. Academic Press, London and New York.
Mohr H. (1972) Lectures on Photomorphogenesis. Springer-Verlag, Berlin.
Mohr H. (1974) Advances in phytochrome research. In Photochem. Photobiol. (in press).
Satter R.L. & Galston A.W. (1975) The physiological functions of phytochrome (in press).
Siegelman H.W. & Butler W.L. (1965) Properties of phytochrome. Ann. Rev. Plant Physiol. 16, 383–93.
Smith H. (1970) Phytochrome and photomorphogenesis in plants. Nature227, 665–8.
Smith H. (1975) Phytochrome and Photomorphogenesis. McGraw-Hill, U.K. Ltd., Maidenhead.