Chapter 13—
Hormone Action
13.1—
Introduction
The genesis of hormone research in plants occurred with the discoveries of Charles Darwin and his son Francis, who published, almost a century ago, their book entitled The Power of Movement in Plants'. Their experiments dealing with phototropism revealed that an 'influence' produced in the tip of a canary grass coleoptile was translocated to the basal portion where it caused the organ to grow towards the light. Almost fifty years later Went isolated a substance with just these properties which was produced in the tip of coleoptiles and transported to the basal portion of the organ. This substance was named auxin. Ten years after Went's (1934) discovery, the structure of this material was identified as indoleacetic acid (IAA).
The nature of auxin effects on plant growth and development was studied in many laboratories in the late 1930's and 1940's. However, it was not until World War II that the selective action of indoleacetic acid served as a basis for developing new chemicals for the control of growth in specific plants. At that time phenoxyacetic acids were discovered and their derivatives were carefully studied under the cover of military secrecy, both in the United States and England. The results of those studies were first published in scientific journals in 1945 and 1947. Today the most widespread uses of growth regulators are as herbicides.
Plant hormones may be divided into five general groups. Auxins include the native indoleacetic acid, as well as the synthetic phenoxyacetic acids, notably 2,4-D (2,4-dichlorophenoxyacetic acid). The second group is the gibberellins which are steroid-like compounds comprising over forty different structures. The third group, the cytokinins, are all N 6 substituted adenosine compounds. The fourth class is represented by a single substance, abscisic acid, which is also derived from isoprenoid units as are the gibberellins. Ethylene, the simplest of all the plant hormones, is a gas and therefore is easily spread from organs of production to organs of sensitivity.
It is interesting that of these five classes of hormones, two are generated from mevalonic acid. Ethylene is produced by the metabolism of methionine and indoleacetic acid is produced by the removal of carbon and nitrogen from tryptophane. Cytokinins, in terms of synthesis, are probably the most complex hormones. They are possibly produced from the breakdown of transfer RNA (tRNA). In the intact tRNA, the adenosine moiety of the RNA is modified by the addition of an isoprenoid group; subsequently, the tRNA is probably degraded, yielding isopentenyl adenosine, (IPA) the endogenous cytokinin.
One might reasonably imagine that an understanding of the physiological role and biochemical mechanisms of action of the plant hormones would have been elucidated quite some time ago. This of course, is in relation to what is known about the many and complex hormones in animals. Unfortunately, however, the plant hormones are not as well understood. Probably the main obstacle to the elucidation of the action of plant hormones has been the fact that they have overlapping and complementary effects on the actions of each other.
During the years between 1960 and 1970 substantial progress appeared to be made on the biochemical mechanisms of action of plant hormones. Much of this research dealt with the effect of the hormones on nucleic acid metabolism, protein synthesis and the synthesis of specific enzymes, since it was felt that the mechanism of hormone action would involve the control of the production of messenger-RNA (mRNA) and the de novo synthesis of enzymes. In the late 1960's the general nature of the research was radically changed because many investigators had found that the plant hormones mediated an effect on cell growth within a few minutes. This information implied a very rapid primary response mechanism of hormone action; consequently, these effects could not be mediated by changing the rate or type of synthesis of nucleic acids or of specific proteins. Many years of research have since been devoted to studies dealing with short-time growth responses to plant hormones. It now seems most likely that plant hormones regulate plant growth through both short-term and long-term growth controls.
It is fairly well understood that animal hormones first interact with the animal cell by binding to, or reacting with, a receptor site of some type within the target cell. Plant physiologists and biochemists are now beginning to consider that the action of many of the plant hormones similarly involves their binding to receptor agents which in turn amplify the action of the hormone and thereby bring about specific changes in nucleic acid synthesis, protein synthesis, enzyme activity and possibly other physiological responses, such as changes in permeability of membranes.
This chapter will summarize the historical evidence which has led to the current level of understanding of the action of plant hormones. Emphasis is placed on the reactive sites within target cells and how the interaction of the hormones with their specific receptors may regulate the growth and development of plant cells.
When a hormone is applied to a responsive plant system it brings about a specific change which results eventually in a measurable biochemical or physiological effect. Two distinct aspects of the measured effect are involved: the specific change in metabolism and the series of steps which lead to the physiological effect. Usually, the molecular interaction of the hormone at its site of action is referred to as the mechanism of action. The subsequent sequence of reactions leading to the physiological effect is referred to as the mode of action. Therefore, by definition each hormone has its own distinctive mechanism of
action even though the manifestation of the hormone mechanism may depend upon prior action by other factors. Thus, it is possible for the hormonal mechanisms in one plant system to lead to a series of physiological responses which may be completely different from those in a second system. This difference in mode of action can be brought about because the second system has more or less of another hormone, has other biochemically rate-limiting components or possesses structural and cytological differences.
13.2—
Auxin Actions
13.2.1—
Introduction
Amongst plant growth regulators, the auxins have been studied the longest and have involved the greatest amount of research. This class of hormones is known to promote cell enlargement or cell elongation, a process which requires extension of the cell wall. Figure 13.1 shows the chemical structures of the major natural and synthetic auxins.
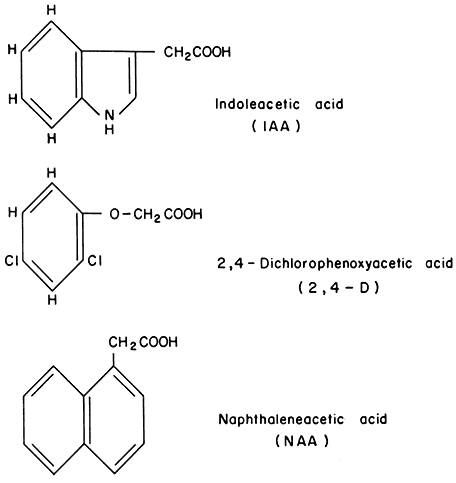
Figure 13.1
Structure of various auxins.
Commencing in 1940, the majority of studies involving auxins were begun in an attempt to determine the molecular interaction with the plant cell. Most of this research was directed toward the cell wall because it was believed that auxin
action required a change in the cell wall to allow for cellular expansion. Thus, the effects of the hormone were thought either to change the cell wall deposition or to hydrolyse certain cross-linkages in cell walls making them more elastic.
This type of research was carried out for several years without achieving a clear understanding of how auxin might control such processes within the cell wall. In the 1950's a new area of research was begun. Under the direction of F. K. Skoog in Madison, Wisconsin, a report (Silberger & Skoog, 1953) was published which showed that the auxin, indole-3-acetic acid, remarkedly affected the RNA and DNA contents of plants. Auxin increased the content of nucleic acids in tobacco tissue cultured on a sucrose-agar medium. This increase occurred prior to the auxin-induced growth of the tissue at concentrations of IAA which were optimal for cell enlargement. For many years following this discovery much research was devoted to the general mechanism by which auxin increased the synthesis of nucleic acids.
A great share of this research was begun in J. Hanson's laboratory in Urbana, Illinois by West and Key (West et al., 1960) who showed that the synthetic auxin, 2,4-D, (see (Fig. 13.1), produced a wide range of morphological and physiological changes in the hypocotyl of soybean and the mesocotyl of corn.
Within 15–24 hours after 2,4-D treatment, cellular enlargement was noted concomitant with an increase in the size of the nucleus. Accompanying these changes were dramatic increases in RNA content, most of which were due to increased ribosomal-RNA. Chrispeels and Hanson (1962) suggested that auxin acts on the nucleus causing it to revert to a meristematic type of metabolism. The role of the nucleus in such a sequence of events is of obvious importance since the nucleus has been shown to accumulate RNA in response to auxin. Beginning in the 1960's, Key began experiments on auxin regulation of nucleic acid synthesis. He and Ingle (1964) demonstrated that auxin controls the synthesis of nucleic acids other than that of ribosomal-RNA. Their data revealed that auxin causes production of nucleic acid which appears to be of the messenger-RNA type.
Subsequent experiments by O'Brien et al., (1968) showed that treatment of soybean with auxin caused a large increase in chromatin-directed RNA synthesis. It was of interest to note that auxin-induced RNA synthesis produced a type of RNA which was different from the control RNA as judged by molecular size and nearest neighbour analysis. Following this area of research other studies (Hardin & Cherry, 1972; Hardin et al., 1970; Hardin et al., 1972) showed that 2,4-D increased the activity of RNA polymerase. Current research suggests that particular cytoplasmic or membrane bound factors may enhance the activity of RNA polymerase. It is hypothesized (Hardin et al., 1972) that the mechanism of action of auxin is to bind to a receptor molecule located on the plasma membrane. The receptor is then released and moves into the nucleus where it modulates the activity of the RNA polymerase. The increase in RNA polymerase activity leads to increased synthesis of messenger-RNAs, which in turn regulate, or control, the synthesis of specific proteins within the target cells.
A primary challenge is to isolate the factor which binds to, or reacts with, auxin, and determine whether this is the primary action of the hormone. Secondarily, research is needed to determine what series of events this interaction puts into metabolic play. It is likely, once the auxin has bound to the receptor molecule within the target cells, that the changes in nucleic acid synthesis cause an increased activity of the various enzymes associated with the cell wall. Many other activities which have been measured over the many years are probably secondary effects resulting from the primary action of the auxin reacting with its receptor molecule.
13.2.2—
Regulation of Cell Wall Extension Ability
When plant cell walls elongate, an accompanying increase in weight and volume also takes place. This increase in size requires that the cell wall be increased in mass as well as area. Even though the dry weight of wall material greatly increases due to cellular enlargement, the thickness and density of the wall remains constant. Thus, wall synthesis appears to be a fundamental requirement during cell elongation. In addition, an increase in wall mass must come from a deposition of polysaccharides. It was believed for many years that auxin, in this particular case indoleacetic acid, increased cell elongation by promoting cell wall synthesis. Auxin, in order to allow for the increased wall extensibility, may also regulate the activity of enzymes involved in cell wall loosening which catalyse the breaking of various cross-linkages between the wall microfibrils.
Plant tissues which respond to auxin by an increased rate of cellular elongation also exhibit an increase in cell wall loosening. However, the kinetics of auxin-induced wall loosening vary considerably from tissue to tissue. For example, in dark grown maize coleoptiles wall loosening is induced by concentrations of auxin around 10–4M , and the increase in loosening proceeds very slowly with time. However, the same tissue responds to auxin very dramatically as maximal increases in total length occur between two and six hours after exposure to auxin. It is of interest, therefore, that the rate of cell wall elongation, as measured by increased section length, is much greater than the increase in cell wall extensibility.
Thus, considerable changes in cell wall extensibility in a given tissue as caused by auxin, may affect growth in a different manner. In certain tissues the rate of growth is constant whilst total wall extensibility changes. In other tissues wall extensibility remains constant during a period of changing growth rate. At the present time it seems that auxin causes a biochemical change in the wall probably by breaking or modifying the cross-links between the wall polysaccharide chains. These changes in the cell wall are then translated under cell turgor pressure into wall elongation. It is now relevant to discuss how auxin affects the cross-links in a cell wall and what the biochemical changes are which take place in a cell wall during cell elongation. In this regard, it is necessary to look at the effect of auxin on various enzymes associated with the cell wall in the context of how these enzymes affect cell wall loosening properties.
13.2.3—
Action on Cell Wall Associated Enzymes
The most dramatic auxin effect on enzyme formation is the induction of cellulase, polygalacturonase and other hydrolases in pea cotyledons treated with a high concentration of IAA (Datko & Maclachlan, 1968). Detection of in vitro formation of cellulase by a ribosomal preparation from peas has been claimed. This was reported to be enhanced using a ribosomal system prepared from IAA-treated peas (Davis & Maclachlan, 1969). The large IAA effect on cellulase formation in vivo is a slow response occurring over several days and cannot be involved in a rapid auxin action on elongation. However, it could be a cause of the lateral swelling response seen in pea cells following IAA treatment.
Auxin stimulates not only the synthesis of cellulase but also the level of glucan synthetase. Masuda (1968) and Masuda and Yamamoto (1970) found that the fungal b -1,3-glucanase, isolated from cultures of Sclerotinia libertans induced rapid elongation of excised oat coleoptile segments. This enzyme was shown to increase cell wall extensibility as measured by a stretching method. In other studies, Masuda et al. (1970), compared the activity of the endo-b -1,3-glucanase and its activity on Avena coleoptile cells with those of the exoenzyme. They found that exoglucanase enhanced elongation and extensibility of the cell wall. But the effect was not additive to the effect of IAA. Furthermore, at least three hours of incubation with exoglucanase was required for enhancement of elongation. The endoglucanase showed no effect on cell wall elongation. Cell wall turnover and auxin effects thereon in pea stem tissue have been studied using pulse-chase wall labelling experiments. Considerable turnover of galactan occurs but this is not influenced by IAA (Labavitch & Ray, 1974).
The action of auxin on soluble xyloglucan can be assayed with relative ease and precision and has been positively demonstrated to be in progress within thirty minutes after exposure to IAA and probably within fifteen minutes, placing it amongst the most rapid metabolic effects of auxin. This auxin effect is blocked by metabolic inhibitors that are known to block elongation, but persists under complete osmotic inhibition of elongation by mannitol. It seems likely that it is involved in the action of the cell wall that leads to elongation in pea cells. Involvement of xyloglucan is understandable in terms of a recent model for cell wall structure, according to which xyloglucan serves to bind matrix polysaccharides to cellulose microfibrils (Bauer et al., 1973; see also chapter 1).
13.2.4—
Hydrogen-Ion Pump
Since the 1930'S it had been known that acidic media can stimulate elongation in auxin-sensitive tissue. Recent work showed that acidic buffers or CO2 solutions in the pH range from 3 to 4 induce a rate of coleoptile cell elongation as great as or greater than that obtainable with auxin (Rayle & Cleland, 1970; Hager et al., 1971; Evans et al., 1971). Acid-induced elongation starts almost immediately upon treatment rather than after the 10–15 minute latent period
characteristic of auxin action. Acid-induced elongation is not suppressible by metabolic inhibitors, such as cyanide, mercurials, and cycloheximide, or by lack of oxygen, all of which block auxin-induced growth, and appears to be a passive process independent of metabolism. In later work it was found that when the epidermis is removed to improve H+ entry into the tissue, the full acid-pH stimulation of elongation develops over the pH range from about 6.0 to about 5.0 (Rayle, 1973; Cleland, 1973).
Rayle et al., (1970b) found that cell wall skeletons of frozen-thawed, dead coleoptile tissue would elongate dramatically if treated with acidic media while being held under tension by an applied load. Rayle and Cleland (1972) concluded that acid pH- and IAA-induced elongation must occur by the same mechanism, because the two kinds of elongation had similar rate, similar temperature dependences, and a similar yield threshold. While suggestive, these similarities do not establish identity of biochemical mechanism.
Hager et al., (1971) also felt, on grounds that IAA-induced elongation could be suppressed by alkaline media, that IAA-induced growth involves the same biochemical mechanism as acid-induced elongation. In support of their proposed auxin-stimulated H+ pump they offered experiments showing rapid stimulation of coleoptile elongation by ATP, ITP, and GTP under anaerobic conditions, although these results may have represented merely the acid pH effect itself, since these compounds are strong acids and were applied in unbuffered, pH 5 media.
In recent work an auxin-induced release of H+ ions from coleoptiles stripped of their epidermis (Cleland, 1973; Rayle, 1973) and from other auxin-sensitive tissues (Marrè et al., 1973; Ilan, 1973) has been detected. This is measured simply as a gradual fall in pH of the medium bathing the tissue, upon treatment with IAA, from near-neutral pH to pH 5 or below, i.e., into the range that by itself induces elongation. Detectable H+ secretion by coleoptiles begins within 20 to 30 minutes after exposure to IAA and may be regarded as a 'rapid response'. To the extent so far studied, H+ secretion by coleoptiles has a specificity for auxin analogues and antagonists similar to the specificity seen in growth, and a similar dependence on metabolism. Secretion is sensitive to inhibitors and uncouplers of energy metabolism and, perhaps unexpectedly for a transport process but just like cell enlargement, H+ secretion is quickly inhibited by low concentrations of cycloheximide (Rayle, 1973; Cleland, 1973). Comparable results with pea stem segments have been reported by Marrè et al., (1973).
These findings, coupled with the observations that induction of elongation by auxin is prevented by sufficiently well-buffered media of pH 6 to 8 provided the cuticle is removed or rendered permeable by gentle abrasion (Rayle, 1973), constitute presumptive evidence that the auxin effect on cell enlargement is mediated by externally secreted H+ ions.
The simplicity and directness of the acid secretion theory of auxin action is appealing and the theory has quickly become popular. Various auxin effects on cation and anion transport noted previously were considered by their authors
to be consistent with the H+ secretion theory. H+ secretion could involve either a parallel flow of anions, or a counterflow of, or exchange with, cations as has been inferred for H+ pumping by beet cells (Poole, 1973). However, as yet no immediate dependence on external ions of either auxin-induced H+ secretion or growth has been found.
Cleland (1973) and Rayle (1973) observed that auxin-treated coleoptiles cease to release H+ when the medium reaches pH 5.0 or slightly below, suggesting that auxin-stimulated H+ secretion is inhibited by acid pH, like other H+ pumps (Poole, 1973), or alternatively that, at pH 5, back-diffusion of H+ into the cell offsets the action of the pump. This feature should allow extensionpromoting pH values in the cell wall to be reached rapidly under auxin treatment, while shutting down further acidification which would probably be injurious to the cell (Cleland, 1973).
Some objections to the H+ secretion theory of auxin action and weaknesses in the evidence for it should be mentioned. For example, it has not yet been shown that suppression of auxin elongation by neutral buffers is not due to inhibited uptake of auxin, or to failure of primary action (the auxin binding found by Hertel et al., (1972) had a pH optimum below pH 6.5).
The most important piece of evidence still needed for the acid secretion theory is that the pH in the tissue's free space falls to an extension-stimulating value at the time that rapid auxin-induced elongation commences, i.e., within 10–15 minutes after exposure to auxin, as against the hour or more that is required for an external bathing medium to reach pH 5.0 to 5.5 (Cleland, 1973; Rayle, 1973). At the very least it must be demonstrated that IAA induces H+ secretion as quickly as it induces rapid elongation. Existing data fail this test and show an increase in H+ efflux from coleoptiles beginning, at the earliest, 20 or 30 minutes after exposure to IAA (Cleland, 1973; Rayle, 1973). These authors feel that the discrepancy may be attributed to time lags imposed by the free space volume and the diffusion path length for H+ to reach the external medium. A worse timing discrepancy was seen with sunflower hypocotyl (Ilan, 1973; cf. Uhrström, 1969). A more sensitive method for measuring H+ efflux needs to be employed to resolve these discrepancies. Better still, the pH within the free space of the tissue should be measured directly during response to auxin treatment.
13.2.5—
Regulation of Genetic Material
After the discovery of messenger-RNA it was suggested that the regulation of gene action by auxin required the specific control of messenger-RNA synthesis. Using a series of inhibitors, Key (1964) and Key and Ingle (1964) noted that treatment of soybean hypocotyl with 5-fluorouracil (5-FU) inhibited total RNA synthesis by 80% whilst growth proceeded normally. Further studies on the fractionation of nucleic acid from soybean hypocotyls on a methylated albumin Kieselguhr column showed that 5-FU inhibited the incorporation of labelled
adenosine diphosphate into ribosomal-RNA but had little effect on the labelling of messenger-RNA. Additional experiments with soybeans showed that 5-FU did not affect the tenaciously bound RNA whose synthesis is promoted by 2,4-D.
Sen and his colleagues (Roychoudury & Sen, 1964; Roychoudury et al., 1965) showed that nuclei isolated from coconut milk responded to auxin by making more RNA in vitro. On the basis of these observations, it was proposed that auxin directly affected the nucleus and thereby regulated gene expression. It was thought that in the presence of the hormone a greater amount of DNA template was exposed and allowed to be transcribed into RNA. In an attempt to test this hypothesis, Cherry (1967) showed that nuclei isolated from peanut cotyledons did not respond in vitro to 2,4-D at physiological concentrations (10–8 to 10–6 M ). From many other experiments, Cherry demonstrated that only in approximately 1 out of 10 attempts could nuclei be shown to respond to the hormone in vitro by the synthesis of more RNA. In general, absolutely no effect of the auxin on the capacity of the isolated nuclei to produce RNA was found. On the other hand, it was found routinely that isolated nuclei from soybean seedlings pretreated with 2,4-D produced twice as much nucleic acid as did control nuclei. Furthermore, experiments of O'Brien et al., (1968) showed that chromatin isolated from soybean hypocotyls did not respond in vitro to 2,4-D. In all cases the tissues needed to be treated with the growth regulator for at least two hours before any effect on chromatin-directed RNA synthesis could be noted. It is to be noted, however, that in a few experiments an effect of the hormone could be observed within thirty minutes. Subsequently, a progressive increase in chromatin-directed RNA synthesis was noted as a function of time. From those experiments, it was suggested that the auxin-enhancement was a result either of a more active RNA polymerase or of gene derepression leading to an increased availability of DNA template. A third possibility was that both of these effects were involved. Subsequent experiments demonstrated that in the presence of E. coli RNA polymerase, chromatin from both control and 2,4-D treated plants allowed the synthesis of similar amounts of RNA at saturation with the polymerase. Even though the total E. coli RNA polymerase activity with chromatin from 2,4-D treated plants was slightly higher than that with control chromatin, it was concluded that the auxin primarily promoted the endogenous RNA polymerase, rather than the chromatin template availability.
When it became possible to solubilize RNA polymerase from chromatin (Hardin & Cherry, 1972) two important aspects were noted. First of all, it has not been possible to show that the addition of auxin in vitro to solubilized RNA polymerase, or to chromatin, increases the rate of RNA synthesis (Hardin et al., 1970). Secondly, of the many experiments that have been performed, it appears that treatment of sensitive plants with auxin leads to a greater production of RNA polymerase I (Hardin & Cherry, 1972; Hardin et al., 1972). This is the enzyme which is thought to be present in the nucleoli, as judged from comparisons with animal RNA polymerase, and is the enzyme thought to transcribe ribosomal into ribosomal-RNA. These data agree with the fact that large increases
in ribosomal-RNA and ribosomes are observed after auxin treatment. However, they are inconsistent with the idea that auxin increases the activity of the RNA polymerase enzyme which transcribes unique DNA sequences into messenger-RNA.
13.2.6—
Action on Membranes
A current, interesting view of auxin action is that the hormone rapidly effects its action at, or on, cellular membranes, possibly involving the regulation of the export of growth active materials across the plasma membrane into the cell wall space. Auxin action at the plasma membrane was hypothesized by Hertel and Flory (1968) and by Rayle et al. (1970) partly in the belief that auxin transport and auxin action on growth involve closely related, if not identical, interactions, presumably with some carrier site located in the plasma membrane. Evidence for auxin action at the plasma membrane comes from observed IAA effects on membrane potentials (Tanada, 1970). This effect, however, has not yet been adequately studied nor shown to be related to the elongation process. Another indication of auxin action at the plasma membrane is the induction of cell elongation by certain fungal toxins which are known to increase membrane permeability (Evans, 1973).
It is assumed that specific auxin receptor sites must be located in or on the plasma membrane if the hormone is to change the permeability or to change any physical property within the membrane. With this general assumption in mind, Lembi et al. (1971) began to look at the binding of labelled NPA (naphthylphthallamic acid) a powerful competitive inhibitor of polar auxin transport which was considered to be likely to bind to specific auxin binding sites. Using maize particle preparations, they tentatively showed that there was a greater amount of NPA binding to fractions rich in plasma membranes in comparison to all other fractions obtained. IAA did not compete with NPA for the sites which thus do not seem to be the actual carrier sites for polar transport of IAA. It is of course conceivable that the sites binding NPA are not the same sites which would normally bind the auxin, IAA.
Hertel et al. (1972) detected a specific binding of labelled IAA and naphthaleneacetic acid (NAA) to certain particles in maize homogenates. Specific binding was assayed as radioactivity retained by particles exposed to 2 to 10 × 10–7 M labelled auxin minus radioactivity retained when, in addition, 10–4M unlabelled IAA or NAA was added to compete with and displace labelled auxin from saturateable binding sites. The radioactivity which remained in the latter case then was termed unspecific binding. This difference-assay gave specific binding with binding constants in the rage of 10– ; to 10–5M . Specificity of the binding as an auxin phenomenon was indicated by the evidence that analogues of IAA or NAA which are active on growth, and are transportable, can displace labelled IAA or NAA from the binding sites, whereas chemically similar, but biologically inactive, molecules do not compete in the binding assay.
One defect of these data was that 2,4-D, a very active auxin, competed relatively weakly with IAA or NAA in the assay, nor was specific binding of labelled 2,4-D itself detected. Based on the evidence that auxin-specific binding sites are localized in plasma membranes, Hardin et al. (1972) proposed that the reaction of auxin, either the native IAA or the synthetic 2,4-D, with the plasma membrane would probably lead to conformational changes within the membrane, causing the release of a receptor molecule into the cytoplasm. This receptor molecule may or may not be the same binding agent with which the auxin interacts. Nevertheless, the receptor released from the plasma membrane could then travel through the cytoplasm and into the nucleus where we know auxin ultimately increases the activity of RNA polymerase.
From previously published information from animal systems, (Shyamala & Gorski, 1969; Jensen et al., 1968) a good analogy can be drawn with hormone interaction with receptor molecules which then migrate into the nucleus where nucleic acid synthesis is controlled. Following these lines of investigation, Hardin et al. (1972) isolated a plasma membrane-rich fraction in which the plasma membrane was thought to be 70% homogeneous. The addition of plasma membranes to soybean RNA polymerase caused an increase in RNA synthesis in vitro. Subsequently, it was shown that pretreatment of the membranes with 10–7M 2,4-D greatly increased RNA polymerase activity. Still further, when the plasma membranes were pretreated with 2,4-D followed by removal by centrifugation, the supernatant fraction contained the RNA polymerase stimulus. The chemical release of this factor appears to be specific for auxin, i.e., IAA and 2,4-D bring about this release, but a non-auxin such as 2,5-D is totally inactive.
In other studies an attempt was made to determine which of the multiple RNA polymerases were stimulated by the auxin-released factor. It was found that the addition of plasma membrane factor to a semi-purified RNA polymerase preparation enhanced the a -amanitin sensitive polymerase. By analogy with animal RNA-polymerase studies, these data imply that auxin increases the nucleoplasmic RNA poly merase, the enzyme which is thought to transcribe DNA into messenger-RNA. If this is true then it is sensible to believe that auxin, through the release of a receptor molecule, may regulate the transcription of unique DNA sequences through the control of a specific enzyme.
13.2.7—
Summary
Because of rapid auxin responses on plant cells, over the last few years a whole new field of research has begun which relates not only to the gene concept but also to rapid responses. Therefore, the new area of research has dealt with actions of auxins on receptors, membranes and other binding surfaces which could lead to a rapid growth response, as well as a continued and long response involving nucleic acid synthesis and protein synthesis.
The apparent binding of auxin to plasma membrane sites has stimulated interest in the concept that the hormone might bring about conformational
changes within the membrane structure. This in turn might lead to changes in permeability, causing an increased ion flux across the membrane. If a receptor molecule is located on the plasma membrane, a change in conformation of the membrane might bring about a release of the receptor. It has been proposed that this receptor moves into the nucleus of the target cell and once there, increases the activity of RNA polymerase. This, in turn, leads to the synthesis of messenger-RNA which codes for proteins and this brings about the net result of auxin increased growth.
In this particular section the effects of cyclic-AMP on auxin controlled growth have not been covered. When cyclic-AMP was first found to stimulate plant growth, considerable excitement was generated (Solomon & Mascarenhas, 1971). However, that excitement was short-lived, and it now appears that cyclic-AMP has little or no effect on plant cells (Ownby et al., 1975).
A number of enzymes have been shown to be affected by auxin. These include the hydrolases, particularly cellulase, and other enzymes associated with cell wall degradation as well as cell wall synthesis. Whilst these enzymes are affected by the auxins, it is very likely that this result is a secondary effect of the auxin and is not related to the primary mechanism of action. In essence then, it appears that auxins control a multitude of physiological and biochemical responses in plants. It is possible that the primary site of action is localized within the plasma membrane or some surface binding site of the cell. A change at this particular level, either in structure or function, could bring about the release of a factor which moves into the nucleus. As a secondary amplification, the hormone thus modulates the activity of RNA polymerase. This in turn leads to the synthesis
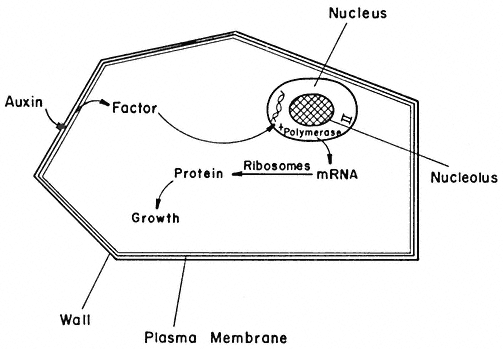
Figure 13.2
A hypothetical model of auxin action. The interaction of auxin with the
plasma membrane results in the release of a factor which moves through
the cytoplasm and into the nucleus. The factor controls the activity of RNA
polymerase II in the nuclei which leads to synthesis of mRNA. The new species
of mRNA translated in the cytoplasm into new proteins leads to enhanced cellular growth.
of RNA from parts of the genome which were either not transcribed at all, or just to a low level in the absence of the receptor. A change in DNA transcription in this manner would lead to the synthesis of new proteins which would bring about the change in the growth potential (Fig. 13.2).
13.3—
Gibberellin Action
13.3.1—
Introduction
Gibberellins may be defined as compounds having a gibbane skeleton and biological activity in stimulating cell division or cell elongation or both (various structures are given in Fig. 13.3). Gibberelins also may possess other biological
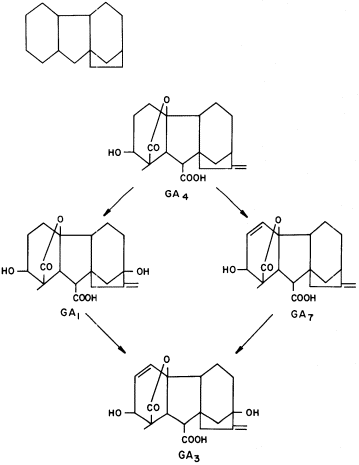
Figure 13.3
Structure of gibbane and the conversion
of GA4 to common gibberellins.
activities such as induction of nucleic acid and enzyme synthesis. Presently there are more than forty known gibberellins which have been isolated from green plants or the fungus Gibberella fujikuroi (Cherry, 1973). Since the late 1960's three to four new gibberellins have been identified each year. Thus, it seems that great progress is being made in the identification of naturally-occurring gibberellins; on the other hand the mechanism of action of gibberellins is still unknown. Plant physiologists are therefore confronted with a very wide array of gibberellins, several of which often occur in the same tissue, and the question arises as to their function within the cells.
Work beginning in the 1960's on the possible regulation of gene transcription by gibberellins was initially successful and achieved wide-spread acceptance. Later work raised many doubts, however, and at the present time, many workers in the field reject the concept that gibberellins regulate physiological processes through the control of gene transcription. Alternative mechanisms involving an action of gibberellins on lipids and membranes are now being proposed.
13.3.2—
Gibberellin Control of Enzyme Synthesis
The aleurone layer of cereal grain is composed of a relatively homogeneous population of non-dividing cells which respond as a target tissue to gibberellins in the concentration range of 10–7 to 10–10 M (Varner & Johri, 1968). It is, therefore, an ideal tissue for a study of the hormonal control of enzyme activity. Haberlandt in 1890 first discovered the secretion of starch liquifying enzymes by the aleurone layer in rye grain in response to some substance obtained from the germinating embryo. Since that early discovery, over the many years following, many workers have found embryo substances or factors and gibberellin-like materials which bring about an increase in activity of several hydrolase enzymes (Varner & Johri, 1968). Some of the enzymes which have been shown to increase in activity in the aleurone layers of barley grains in response to treatment with gibberellic acid (GA3 ) include the following: a -amylase, proteinase ribonuclease, b -glucanase, and pentosanases. A similar control of amylase activity by gibberellins has been observed in wheat and in wild oats. In barley aleurone layers GA-induced increase in a -amylase is prevented by the lack of oxygen, by cytochrome c inhibitors and inhibitors of RNA and protein synthesis. Varner and Johri (1968) have shown that gibberellin control of amylase appears to involve the control of nucleic acid and protein synthesis.
GA3 enhances the development of a -amylase activity in cereal grains. The enzyme is synthesized de novo and its synthesis blocked by cycloheximide and other inhibitors of protein synthesis. It is of interest to note that actinomycin D (an inhibitor of RNA synthesis) only inhibited the production of a -amylase when the tissue was exposed to the inhibitor early in the incubation process. If, however, the tissue was exposed to actinomycin D at some point after enzyme
synthesis had begun, little or no inhibition occurred. Thus, it was not certain whether the hormone actually controlled the synthesis of RNA at the level of transcription or controlled the translation of pre-existing messenger-RNA. Many attempts were initiated to answer this question; unfortunately, to date, a definitive decision has not been possible. The most likely conclusion is that the hormone does not control the synthesis of a -amylase at transcription (Chrispeels & Varner, 1967).
13.3.3—
The Effects on RNA Synthesis
Considerable indirect evidence has shown that GA3 influences the synthesis of RNA in many plant systems (Varner & Johri, 1968). If this RNA synthesis is a primary effect of the reaction of GA3 within a cell, it is then most likely that the primary action occurs in the nucleus. In many other plant tissues also, either an increase in protein and RNA synthesis or in the incorporation of labelled precursors into these macromolecules in the target tissues is evoked by hormones (Key, 1969).
It seems obvious that if GA interacts directly with the nucleus it would be advantageous to work with isolated nuclei or chromatin obtained from the nuclei. Therefore, attempts were made to work with nuclei isolated from light-grown dwarf peas (Varner & Johri, 1968). To determine if the RNA synthesized in the presence of GA3 was qualitatively different, the nuclei were incubated in the presence of one of the four nucleoside triphosphates labelled in the a position with 32 P and the radioactivity then determined in the 2',3' nucleotides. It was found that a significant difference existed in the relative distribution of radioactivity among the four nucleotides of the RNA synthesized in the presence and absence of gibberellic acid. This nearest-neighbour analysis indicated that RNA synthesized by GA-treated nuclei is in part qualitatively different from the RNA synthesized by untreated nuclei.
Fractionation of the labelled RNA on MAK columns showed differences also in the types of nucleic acids produced in the presence of GA3 . The results indicated that GA preferentially enhances the synthesis of a small fraction of RNA. However, fractionation of the labelled nucleic acids on sucrose gradients indicated that the in vitro synthesized RNA sediments in a peak corresponding approximately to 6–10s. These results indicate that the RNA synthesized by GA-treated nuclei has a higher average molecular weight than that synthesized by the control nuclei. Varner and Johri (1968) concluded that the enhanced RNA synthesis could be due (a) to an increase in template DNA availability, (b) to an increase in the RNA polymerase activity, or (c) to a combination of the above two possibilities. An enhanced activity of RNA polymerase could be due to activation or to protection against degradation of the enzyme. Another explanation might be that the hormone changes the permeability of the nuclear membrane and thereby makes the RNA precursors more available for use in transcription.
13.3.4—
Gibberellin Control of Enzyme Secretion
The gibberellin-enhanced synthesis of hydrolases is preceded by a proliferation of rough endoplasmic reticulum (Yomo & Varner, 1971). The control of rough endoplasmic reticulum seems more likely than the control of hydrolase synthesis and secretion as a candidate for the primary site of gibberellin action since it could more readily be a general response of plant tissues to gibberellins. Varner and his associates (Evins & Varner, 1971; Koehler & Varner, 1973) have therefore examined rough endoplasmic reticulum in detail. There is a gibberellinenhanced incorporation of labelled choline into the lipid-soluble material of the endoplasmic reticulum and of other cell fractions. An enhanced incorporation of 32 P into the phosphoryl-lipids of several cell fractions is also seen. This enhancement is first measurable about four hours after the addition of gibberellin and is proportional to the logarithm of the concentration of the hormone.
GA3 also enhances the activity of phosphorylcholine cytidylate-transferase and phosphorylcholine-glyceride transferase (Johnson & Kende, 1971). These responses are measurable as early as two hours after treatment. Gibberellin-evoked increases in these membrane-bound enzyme activities are prevented by the inhibitors, abscisic acid, actinomycin D and cycloheximide. These early effects on phosphoryl-lipid metabolism suggest that hydrolase synthesis occurs only on polysomes bound to newly synthesized endoplasmic reticulum. Thus the synthesis of hydrolase before the addition of GA3 may be limited not by messenger-RNA but by the availability of appropriate membranes for the attachment of polysomes which carry hydrolase-specific messenger-RNAs. An examination of the secretion of hydrolases is of interest because it appears that gibberellin is required for the secretion of b -glucanase which had been synthesized before hormone addition. Gibberellin-enhanced release of b -glucanase into the medium surrounding the aleurone cells is prevented by inhibitors and uncouplers of oxidative phosphorylation, by actinomycin D, 6-methylpurine, and by cycloheximide. This is in contrast to the secretion of a -amylase which requires phosphorylative energy transformation but is not affected by 6-methylpurine nor by cycloheximide. These differences in the secretion of b -glucanase and a -amylase may represent different modes of secretion for the two enzymes. Or, it may be that the cellular apparatus required for the secretion of both enzymes is made during the first few hours of gibberellin treatment.
13.3.5—
Effects of Gibberellins on Membranes
For the past few years Paleg and his associates have studied the effects of GA3 on various membranes and membrane properties (Wood & Paleg, 1974; Wood et al., 1974). Their studies were initially encouraged by the work of Bangham et al. (1965) who provided evidence that steroids have potent effects on model membranes composed only of lipids. Correlation between steroid activity in vivo
and in the model membrane systems was good. A suggestion was made that steroids produced their biological effect through a direct interaction with lipid, independent of polysaccharides, proteins or even active cell metabolism. The influence of GA3 on the permeability of lipid model systems was first described by Wood and Paleg (1972) who suggested that gibberellins might similarly exert their physiological action through an effect on lipids. Recently, Wood et al. (1974) have demonstrated complex formation between GA3 and phosphatidyl choline thus providing definitive evidence of a molecular association between the hormone and a common membrane component. In this study, complex formation was dependent on hormone concentration. Wood and Paleg (1974) also showed that the process of glucose diffusion from liposomes (i.e. microvesicles composed of a bimolecular lipid membrane surrounding aqueous contents) is not affected by the presence of GA3 . The same energy is required for a mole of glucose to permeate the lipid at each temperature in the presence or absence of the hormone. However the barrier to diffusion is perturbed in such a way that more glucose can pass per unit of time in the presence of GA3 . Furthermore, the temperature at which the lipid membrane undergoes a change in conformation (the transition point) is lowered by several degrees by hormone treatment.
By comparison with living systems, GA3 could, by its influence on lipid polarity, affect two main processes in vivo; (a) the permeability of the membranes to regulatory ions which act as limited substrates; and (b) the activity of membrane-bound enzymes such as ATPase which are activated by sodium, potassium, etc. Both of these effects could be brought about by the same mechanism—greater membrane fluidity. Since GA3 influences not only the transition point at which the liposome changes in structure but also the diffusion rate of glucose above this transition point, one or both of two mechanisms may explain its action. Either its presence directly reduces the viscosity of the central core of the membrane or, more likely, it perturbs the still-ordered polar regions of the liquid crystal phase leading to greater fluidity.
However, the temperature zone of greatest physiological interest is that where the untreated system is below the transition point while in the presence of hormone the system has switched to high temperature sensitivity. The relationship between biological activity of different gibberellins and their ability to influence the thermal transition and membrane permeability has not been explored extensively. However, the methyl ester of gibberellic acid, a derivative with only about 10–3 of the biological activity of unsubstituted GA, was found to be without effect on the temperature at which the transition of the membrane occurs. In this regard it is of interest that it also induces very little alteration of the nuclear magnetic resonance spectrum of lecithin, which indicates little complex formation between the hormone and the fatty acid. This new area of research may suggest that the mechanism of GA action is through the direct alteration of membrane structure and/or function.
13.3.6—
Summary
The gibberellins comprise a complex and large group of related compounds which control cell elongation and enzyme secretion. The mechanism by which the hormone turns on the synthesis of enzymes has been studied in fairly close detail. Initially, it was felt that the hormone regulated the synthesis of RNA at the level of transcription. Through the careful and dedicated work of Varner and his associates it appears reasonably clear that the hormone does not control the secretion of enzymes by controlling the synthesis of messenger-RNA. However, it is clear primarily from the work on amylase, that inhibitors of nucleic acid synthesis and protein synthesis disrupt the production of the enzyme. The recent experiments of Paleg and his associates bring a new dimension to the question of gibberellin action by their demonstration that GA3 affects the membrane permeability of fluidity. Thus, a new range of possible hormonal mechanisms can now be envisaged and explored.
13.4—
Action of Cytokinins
13.4.1—
Introduction
The term cytokinin has been accepted practically universally as a generic name for substances which promote cell division and exert other growth regulatory functions in the same manner as kinetin. The synthesis and testing of compounds for cytokinin activity began with the discovery of kinetin (6-furfurylaminopurine). Today there are probably at least one hundred known synthetic and native cytokinins (Fig. 13.4).
Structural requirements for a high order of cytokinin activity generally include an adenine molecule with the purine ring intact and with an N6 substituent of moderate size. One exception to the requirement for a modified purine exists, notably diphenylurea and its derivatives. Certain other substances which lack a true purine ring, such as 8-azakinetin, 6-benzylamino-8-azapurine and 6-(3-methyl-2-butenylamino)-8-azapurine are active, but each of them is less than 10% as active as its corresponding purine derivative. Substitution of either O or S for N in the N6 position of adenine in each case results in a more than 90% loss in growth promoting activity in the tobacco bio-assay.
Cytokinins play a role in practically all phases of plant development from cell division to the formation of flowers and fruits. They affect metabolism including the activities of enzymes and the biosynthesis of growth factors. They also influence the biogenesis of organelles and the flow of assimilates and nutrients within the plant. Cytokinins defer senescence and may protect the plant against adverse environments, such as water stress. These many diverse effects presumably stem from some primary anabolic function of the cytokinins that remains to be elucidated.
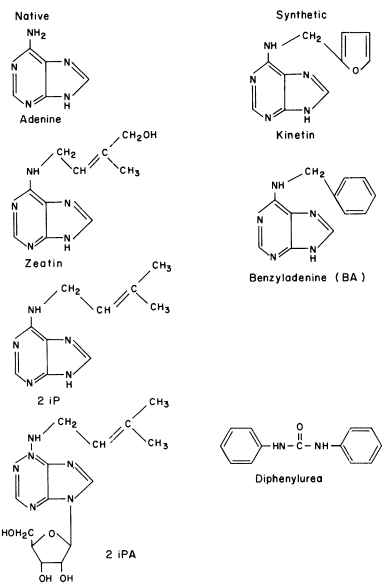
Figure 13.4
Structure of some common
native and synthetic cytokinins.
Cytokinins have been found in certain transfer-RNA (t-RNA) molecules from a large number of organisms including bacteria, yeast, plants and animals (see Cherry & Anderson, 1971). In sequence analysis the native cytokinin, isopentenyl adenine, always occurs adjacent (3' side) to the anticodon (Fig. 13.5). Furthermore, the location of the isopentenyl group is required for the
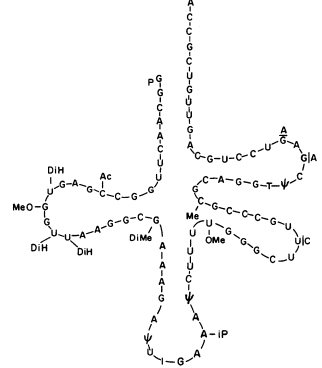
Figure 13.5
Diagram of the cloverleaf shape of seryl-transfer RNA.
The IPA residue is located adjacent to the anticodon.
specific tRNA to function efficiently in protein synthesis. Results involving bacteriophage infection of bacteria indicate that cytokinin in tRNA may have an important regulatory function in protein synthesis. In soybean seedlings (Anderson & Cherry, 1969) the application of a cytokinin (6-benzyladenine) results in increased levels of two species of leucyl-tRNAs. Although the relationship of cytokinins to tRNA may not be directly associated with the primary action of cytokinins on cellular activity, the alteration of specific tRNAs could control protein synthesis. Such a regulatory mechanism would be manifested in the control of growth and morphogenesis as the ultimate expression of the hormone.
13.4.2—
Actions of Cytokinins on Enzymes
Cytokinins have been shown to influence the activities of a number of specific enzymes. A most thoroughly studied case is the promotion by cytokinins of tyramine methylpherase activity in roots of germinating barley embryos. This appears to involve de novo synthesis although this conclusion is based solely on
the results of inhibitor experiments (Mann et al., 1963; Steinhart et al., 1964). Activities of four other enzymes tested were unaffected by kinetin treatment. The selective action on methylpherase is most likely related to the developmental stage of the seedling material and not to a specific affinity of cytokinin for the enzyme. Substances other than cytokinins (auxins and lysine) which also stimulated the appearance of tyramine methylpherase activity either were less effective or acted only during the early stages of germination. An analogous effect of kinetin in promoting synthesis of alkaloids in the roots of lupin has also been reported (see Skoog & Armstrong, 1970).
The induction of a -amylase activity in half-seeds without embryos, a well known effect of gibberellins in barley, is achieved in wheat also by treatment with cytokinins (Boothby & Wright, 1962). However, the effect of cytokinins on wheat is much less marked than that of gibberellin in the barley. In barley seeds, cytokinins cannot replace gibberellins, but do counteract an inhibition of gibberellin action by abscisic acid (Khan, 1969). The formation of amylase activity in excised hypocotyls of pea is stimulated by cytokinins but not by gibberellins or auxins (Chum, 1967). In germinating squash seeds cytokinins, but not gibberellins, at least partly substitute for the embryo in promoting the formation of isocitrate lyase and proteinase activity in the cotyledons.
The synthesis of deoxyisoflavones in soybean callus tissue is promoted by both cytokinins and auxins (Miller, 1969). The ease with which the compounds can be detected and the rapidity of the response suggests that this system might be useful as a rapid bioassay for cytokinins.
The rates of synthesis of ribulose bisphosphate carboxylase and NADP-dependent glyceraldehyde-phosphate dehydrogenase, studied in rice seedlings, appear to be under cytokinin control (Feierabend, 1969). Kinetin did not affect these enzymes until 96 hours after the beginning of germination. By contrast the activity of the cytoplasmic enzyme 6-phosphogluconic acid dehydrogenase was promoted by kinetin only during early stages of germination. It was concluded that cytokinins probably are not directly involved in gene derepression although the level of cytokinin may determine the extent to which the appropriate genes are expressed.
13.4.3—
Nucleic Acid Synthesis
Early studies with tobacco pith tissue showed that a proportion of the tetraploid cells present would be stimulated by kinetin treatments to enter mitosis and undergo cytokinesis without incorporation of thymidine. Thus the effect of kinetin on cell division was not always associated with DNA synthesis. In Lemna cultures 6-benzyl-aminopurine reverses the effect of abscisic acid in inhibiting growth and nucleic acid synthesis (van Overbeek et al., 1967). DNA synthesis in response to these growth substances appears to be more rapid than RNA synthesis. In axillary buds of tobacco which are normally inhibited by
the apex, treatment with 6-benzylaminopurine initiates DNA synthesis and growth (see Skoog & Armstrong, 1970).
Some inhibitors such as chloromycetin and 6-azauracil uncouple the two processes, preventing growth but not the cytokinin-induced DNA synthesis. Nevertheless, in view of the early work, it seems likely that cytokinin effects on DNA synthesis are indirect. In a detailed study of tuberization, Palmer and Smith (1969) have shown that, in excised stolons of potato, cytokinins inhibit stolon elongation and induce tuber formation. Other factors which inhibit elongation may also increase the inductive response in response to cytokinins, but they are not themselves inductive factors. Inhibitor studies suggest that cytokinin action is associated with protein synthesis or activation but not with DNA or messenger-RNA synthesis. Effects of cytokinin on RNA and protein synthesis and isolated cell organelles have been reported, but have not yet been analyzed in depth (Skoog & Armstrong, 1970). Nuclei preparations separated from coconut milk and incubated in the presence of kinetin showed an increased incorporation of labelled precursors into RNA and protein (Datta & Sen, 1965).
The effect of kinetin on RNA synthesis in an in vitro system containing purified chromatin and E. coli RNA polymerase has been studied by Matthysse and Phillips (1969). In the presence of a particular protein fraction, kinetin stimulated RNA synthesis. A similar effect of auxin was also mediated by a protein fraction. The effects of cytokinin in this system have not yet been studied in great detail. Fellenberg (1969) has observed that in vitro bindnig of kinetin to chromatin of pea epicotyls caused a slight decrease in the melting point of the nucleoprotein complex.
13.4.4—
Role of Cytokinins on Transfer RNA
One suggestion has been that the endogenous cytokinins somehow exert their activity by being incorporated into transfer-RNA (Skoog & Armstrong, 1969). For example, the synthetic cytokinin, benzyladenine, was reported to be incorporated into the tRNA of callus tissue (Fox & Chen, 1967). Richmond et al. (1970) specifically looked for incorporation of labelled benzyladenine into the RNA of senescing leaves, but found no evidence for incorporation. On the other hand, under similar conditions kinetin was incorporated into the RNA. Recently Walker et al. (1974) have shown that double-labelled benzyladenine is incorporated into tRNA at a frequency of one per 104 molecules.
Chen and Hall (1969) have shown that cytokinin-dependent tobacco callus tissue contains the enzyme system which attaches the D2 -isopentenyl group to the appropriate adenine residue in tRNA. In the case of tobacco callus tissue grown in the presence of radioactive benzyladenine, the tRNA still contained its complement of isopentenyl adenine derivatives even though the benzyladenine was incorporated into the tRNA. In general, various purine analogues can be incorporated into RNA in a rather non-specific way.
It is also quite possible that incorporation occurs into RNA fractions other
than tRNA, and unless the tRNA is rigorously characterized one cannot exclude the possibility of error arising by contamination with other RNA fractions. To establish whether the incorporation of N6 -substituted adenosine derivatives into tRNA has a biological significance it would be necessary to fractionate the tRNA and identify the incorporated material at a specific location in the tRNA sequence.
13.4.5—
Model for Cytokinin Action
Cytokinins are found in transfer-RNAs of animals, microorganisms and plants (see Cherry, 1973). Furthermore, in those cases where the nucleotide sequence is known the native cytokinin, IPA (isopentenyladenosine), or a modified form, is found adjacent to the anticodon. At the moment it appears that all IPA containing species of tRNA recognize codon triplets which begin with U (Skoog & Armstrong 1970). Thus, the anticodon loop of a cytokinin-containing RNA could be one of at least sixteen codon sequences of the following type: A— —IPA. By eliminating the nonsense terminator and the phenylalanine codons, it seems that IPA-containing tRNAs would be confined to less than ten codons (see Table 13.1).
|
It may be that IPA adjacent to the anticodon of these tRNA species plays some role in messenger-RNA-tRNA-ribosome recognition, and that these tRNA species are required for the translation of some messenger-RNAs into specific proteins. Based on these assumptions it is possible to speculate on the mechanism of action of cytokinins in plants. From the observation that cytokinins appear not to be incorporated into tRNA, as precursor units, but rather the isopentenyl group comes from mevalonic acid (Chen & Hall, 1969), it seems likely that cytokinins have no effect on the synthesis of tRNA containing IPA. Furthermore, a number of chemicals, including various isomers of IPA, kinetin, 6-benzyladenine and even diphenylurea, have cytokinin biological activities, and it appears that these cytokinins do not participate in tRNA synthesis. If this is true, then what is the mechanism of cytokinin action?
A model presented in Fig. 13.6 is based on the speculation that specific
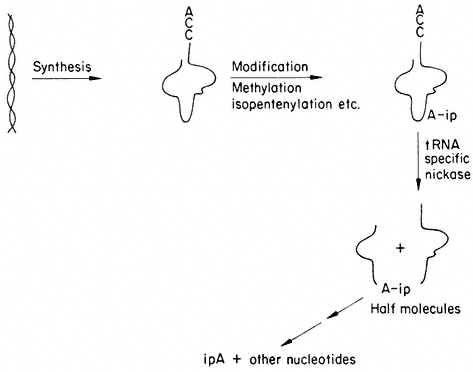
Figure 13.6
A model of cytokinin action. Based on the fact that tRNA specific nucleases
are known, it is suggested that free cytokinins protect cytokinin-containing
tRNAs by inhibiting nuclease activity, specific as well as general.
nucleases break the primary structure of the IPA containing tRNA (Cherry & Anderson, 1971). The IPA would provide a unique attachment for the enzyme to bind to and subsequently to break the phosphodiester bonds. The action of this enzyme would destroy the function of the tRNA and yield free cytokinin. Cytokinins including 6-benzyladenine and diphenylurea added exogenously to plants or tissue cultures would defer senescence by essentially protecting the cytokinin-containing tRNA species. This protection would be mediated by the exogenously added cytokinin binding to the nuclease and competitively inhibiting its action. Alternatively, the cytokinin could bind to other agents which in turn prevent an increase in nuclease activity which would destroy the cytokinin-containing tRNA. Therefore, tissue treated with cytokinin would retain its IPA-containing tRNA species and continue to synthesize essential proteins as long as sufficient free cytokinin was present within the tissue.
Preliminary unpublished work of Locy (1974) provides suggestive evidence of a tRNA-specific nuclease. We have found that chloroplasts of soybeans contain a nuclease which specifically degrades one of the three tyrosyl-tRNAs. At the moment we do not have any evidence to suggest that free cytokinin controls the activity of this tRNA nuclease. In regards to this model it is to be emphasized that it is hypothetical and is presented as a working base upon which to investigate further the mechanism of action of cytokinins at the molecular level.
13.4.6—
Summary
Cytokinins have been shown to increase the activities of a number of enzymes. These increases in activity do not appear to be a direct effect of the cytokinin
but rather to be an indirect effect of some kind. Cytokinins increase the amount of DNA produced in a number of tissues. Furthermore, cytokinins increase the rate of RNA synthesis in ageing tissue. However, a number of studies have pointed out that the cytokinin effect on nucleic acid synthesis most likely is not due to a direct effect on the derepression of the DNA within the genome.
Over the past few years there has been a great deal of excitement generated relating to the fact that the native cytokinin is found in some transfer-RNA isoaccepting species. For a few years data was presented which showed that cytokinins, particularly the synthetic cytokinin, 6-benzyladenine, was incorported directly into transfer-RNA. That excitement, however, appears to be shortlived since other studies have shown that the incorporation of the labelled synthetic cytokinins is not into tRNA species but into heavier forms of RNA. Therefore, it appears quite clear that cytokinins are likely not incorporated into tRNA directly. A model is presented (Fig. 13.6) which suggests that the free cytokinins act as a protective agent within the target cells of plants to protect the transfer-RNA molecules which contain the native cytokinin. It is suggested that there are specific nucleases which degrade only certain species of transfer RNA. The model implies that free cytokinin inhibits the nucleases through product inhibition, or possibly through some other mechanism which prevents the enzyme from attacking the tRNAs which contain the cytokinin. At present there is only a small amount of evidence to substantiate this model.
13.5—
Actions of Ethylene
13.5.1—
Introduction
Ethylene is the simplest hormone which regulates plant growth (Fig. 13.7). It is a natural constituent of plants and affects a wide array of physiological processes. For many years, however, investigations of ethylene physiology were concerned primarily with fruit ripening. With the advent of gas chromatography many experimenters have begun research on the biochemical and physiological action of ethylene. The production of ethylene by germinating seeds and seedlings suggests that the hormone may be involved in the normal regulation of growth and development. Evidence for such a proposal includes the fact that low concentrations of applied ethylene blocks photo-induced apical bud expansion and hook opening in etiolated pea seedlings. It was shown that apical tissues of

Figure 13.7
Structure of ethylene.
etiolated seedlings are the major site of ethylene production. Light has been found to decrease the tendency of pea stem segments to produce ethylene in response to high concentration of auxin.
On the other hand, red light appears to stimulate ethylene production in dormancy. Another important role of endogenous ethylene in etiolated seedlings is the regulation of radial expansion of the pea epicotyl in a region below the apical hook. The exposure of the plant to red light or to CO2 inhibits an ethylene-mediated increase in the diameter of the epicotyl.
Growth, flowering, abscission and fruit ripening all are affected by ethylene. It is currently popular to speculate that the mode of action of ethylene involves a mechanism which regulates some aspect of the transcription of DNA or the translation of RNA. Studies of the effects of ethylene on abscission and growth indicate sizeable changes in RNA and protein contents. There is indeed evidence of changes in the activities of catalase, peroxidase, and other hydrolases. Furthermore a study showed that exposure of soybean plants to ethylene significantly altered RNA polymerase activity associated with chromatin. As judged by nearest neighbour analysis the RNA produced from ethylene treated plants has a different base composition than that from the control. Even though ethylene at very low concentrations affects a wide array of physiological and biochemical processes in plants, much more work is required to know the mechanism of action.
13.5.2—
Effect of Ethylene on Enzymes
A number of investigators have examined the possibility that ethylene has a direct effect on enzyme activity (Abeles, 1973). However, investigations with b -glucosidase, emulsin, a -amylase, invertase, peroxidase, and adenosine triphosphatase have shown that ethylene has no effect on these enzymes. Results reported by Abeles (1973) have similarly shown that ethylene has no effect on cellulase and carbonic anhydrase. Carbonic anhydrase was chosen because it appeared to be a likely candidate to show a positive response. Carbonic anhydrase contains zinc and has the ability to combine with CO2 , both features which suggest potential sensitivities for ethylene action. Nelson (1939) reported that ethylene increased the activity of trypsin. However, this effect was thought to be due to the removal of O2 since H2 had the same effect.
13.5.3—
Actions on Membranes
Since ethylene is more soluble in oil than in water and since membranes contain large amounts of lipid material, a number of investigators have tested the idea that ethylene affects some aspect of membrane permeability. However, proponents of this idea have failed to note that CO, an ethylene analogue, does not share the same lipid solubilities as ethylene but nevertheless has similar physiological activities. The idea that ethylene has a disruptive effect on the membrane
causing a change in permeability and an alteration in compartmentalization does not appear valid. Ripening fruits exhibit obvious changes in terms of permeability and retention of soluble components and it seems natural to suggest that ethylene leads to changes in membrane permeability which in turn causes softening and increased respiration. However, current evidence suggests that a change in membrane properties is an effect of ripening rather than a cause.
A similar situation exists in flowers. Nichols (1968) pointed out that solute leakage increased from carnations during senescence. Senescence and leakage were promoted by ethylene and reversed by CO2 . Ethylene has no influence on membrane permeability of potato, pea, avocado, banana, and bean. However, on the other hand, von Guttenberg and Beythien (1951) reported that ethylene increased the rate of deplasmolysis of

13.5.4—
Enzyme Induction by Ethylene
Regeimbal and Harvey (1927) were the first to report that ethylene-treated tissues contained higher levels of particular enzymes than the control. They found that ethylene increased the level of protease and invertase extracted from pineapple fruits. Since that time, reports (see Abeles, 1973) have appeared which show effects of ethylene on a number of enzymes. This list includes acid phosphatase, ATPase, a -amylase, cellulase, chitinase, chlorophyllase, cinnamic acid 4-hydroxylase, cytochrome c reductase, diaphorase, b -1,3-glucanase, invertase, malic enzyme, pectin esterase, peroxidase, phenylalanine ammonia-lyase, polygalacturonase, polyphenyloxidase, protease and pyruvic carboxylase.
Enzyme induction does not always depend entirely on the action of ethylene. In some cases, cutting the tissue causes an increase in enzyme activity. The function of ethylene is to reduce the lag time or increase the rate of increase in enzyme activity. Examples of enzymes whose increased activity does not strictly depend on ethylene action include b -1,3-glucanase, malic enzyme, phenylalanine
ammonia-lyase and peroxidase (Abeles, 1973). Excising tissue can cause wound ethylene production and it is possible that this source of ethylene plays some role in enzyme induction in tissue slices. On the other hand, enzyme induction during abscission was dependent on ethylene and for a reasonable period of time no cellulase synthesis or abscission occurred following excision unless ethylene was added to the gaseous phase.
13.5.5—
Effects of Ethylene on RNA Synthesis
The first report of ethylene effects on RNA synthesis was by Turkova et al. (1965) who reported an increase in RNA synthesis during epinasty of tomato leaves. Whether or not RNA synthesis was required for epinasty was not shown, although the idea is intriguing. Studies with actinomycin D indicated that RNA synthesis occurred during abscission and was required for the process to occur (Abeles & Holm, 1966). Support for this interpretation stems from the work of a number of investigators (see Abeles, 1973). It is known that the increase in RNA synthesis precedes that in protein synthesis and is localized at or near the separation layer. The increase in RNA occurred in all fractions including messenger-RNA, ribosomal-RNA and transfer-RNA. Differential extraction of the nucleic acids indicated that the ethylene-stimulated fraction was confined to that portion of RNA extracted by sodium lauryl sulphate with the increase found in ribosomal- and messenger-RNAs. The inhibitor, 5-fluorouracil, which blocks 50% of the ethylene-enhanced incorporation of 32 P into RNA did not inhibit abscission. The greatest inhibition occurred in transfer-RNA and ribosomal-RNA which indicates that the synthesis of all fractions of RNA is not required for abscission. Presumably as long as messenger-RNA was being synthesized, enough ribosomal- and transfer-RNAs were already available within the cell to permit abscission to take place. When all RNA synthesis was blocked by actinomycin D, abscission stopped (Holm & Abeles, 1967). Ethylene has also been found to promote RNA synthesis in preclimacteric fruit. Marei and Romani (1971) reported, as in the case of abscission, that the synthesis of all classes of RNA in fig fruit was stimulated. Holm et al. (1971) found that ethylene increased RNA synthesis in apples and that the increase in RNA was followed by an increase in protein synthesis. However, Sacher and Salminen (1969) reported that they failed to find an increase in RNA synthesis when preclimacteric bananas or avocados were treated with ethylene.
13.5.6—
Effects of Ethylene on Chromatin Activity
Holm et al. (1970) have reported that ethylene inhibited the growth of the apical part of soybean seedlings and caused an increase in the elongating and basal portions of the stem. At the same time, RNA levels in the apical zone were reduced while the levels were enhanced in the elongating and basal regions. Chromatin from the various parts of the seedling were studied to determine if the
capacity for RNA synthesis was also modified. They found that the activity in the apex was reduced while the activity in the elongating and basal regions were promoted. The rate of the response was rapid since the increase in chromatin activity was apparent after 3 hours. Nearest-neighbour analysis of the RNA synthesized demonstrated that there was a qualitative difference between RNA synthesized from chromatins of normal and ethylene-treated tissue. It was concluded that ethylene can regulate RNA synthesis as manifested by a change in quantity and kind of RNA.
13.5.7—
Effects of Ethylene on DNA Metabolism
Plant growth is either promoted, inhibited or unaffected by ethylene depending upon the tissue involved. Examples of growth promotion are swelling, epinasty, hook closure, seedling elongation and seed germination (Abeles, 1973). Bud break is probably a special case. Here no growth takes place as long as ethylene is present. However, after the gas is removed growth of the buds ensues. Growth inhibition is seen as arrested development of buds, leaves or apical meristems. Mature tissue, such as stems and leaves. do not undergo any change of size or weight, although premature senescence usually occurs. Since growth, or the lack of it, may be associated with cytokinesis, it is of interest to learn that DNA synthesis is controlled by ethylene. Holm and Abeles (1967) reported that DNA synthesis or DNA content in bean leaf tissue was not affected by seven hours exposure to ethylene, although abscission was promoted. Later they found that in soybean seedlings treated with ethylene the synthesis of DNA was inhibited in the apex where growth was inhibited but was promoted in the subapical part where swelling took place. Burg et al. (1971) found a similar situation in pea seedlings in which inhibition of cell division, measured as a reduction in metaphase figures, occurred within two hours after ethylene was added. However, it is not clear whether the change in DNA synthesis was the cause or the result of inhibited growth. Ethylene slows the growth of pea seedlings very quickly; for example, Warner and Leopold (1971) found growth slowed six minutes after ethylene was introduced into the gas phase and returned to normal sixteen minutes after the ethylene was removed. Kinetic studies on changes in DNA or other postulated sites of action are thus required to establish the relationship between cause and effect. Burg et al. (1971) have suggested that ethylene regulates DNA synthesis by some action on microtubule structure essential for spindle fibre formation during mitosis. If the action of ethylene was directed toward microtubules, this might also explain the reorientation of cellulose microfibril deposition that occurs in swelling.
13.5.8—
Summary
Even though little is known about the binding site(s) of ethylene, in a few cases such as ripening, abscission, swelling and senescence there is reason to believe
that the combination of ethylene with a site results in the regulation of protein or enzyme synthesis which in turn accounts for the observed responses. In these cases RNA synthesis, presumably messenger-RNA, with the accompanying support of soluble- and ribosomal-RNA, is an essential or early step suggesting regulation of gene action. However, in other cases, especially those associated with the physiology of excised tissue, control is probably not exerted at the level of RNA or protein synthesis. In the case of the inhibition of elongation, the action is apparently directed toward blockage of DNA metabolism. The site of action of ethylene in epinasty, root initiation, intumescence formation and floral initiation is even more poorly understood. The only valid conclusion appears to be that a number of essential features of plant growth and development are susceptible to ethylene action. In the final analysis it is concluded that there may be as many mechanisms of ethylene action as there are modes of ethylene operation.
13.6—
Actions of Abscisic Acid
13.6.1—
Introduction
Abscisic acid (ABA) is a plant hormone which now ranks in importanance with the auxins, gibberellins, cytokinins, and ethylene. Interest in the physiology and chemistry of ABA has grown greatly since the structure was established in 1965. During the 1950's and early 1960's a number of laboratories were engaged in research on growth-inhibiting substances. ABA was first isolated from cotton plants and was named abscisin II by a team from Addicott's laboratory (Ohkuma et al., 1963). During the same year Wareing's research team (Eagles & Wareing, 1963) isolated an active substance from Acer leaves which they named dormin. Abscisin II and dormin are the same substance which is now called abscisic
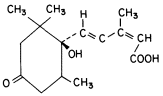
Figure 13.8
Structure of abscisic acid.
acid (Fig. 13.8). Plant tissues of all ages appear to synthesize and inactivate ABA. The number of various plant responses affected by ABA is very large. Generally, the physiological processes are related to senescence or abscission and growth retardation or inhibition. ABA appears to act as an abscission accelerating hormone in many fruits and leaves. Furthermore, it also tends to induce dormancy in some woody plants. ABA has been shown to move from the leaves to the apical bud to bring about a dormant condition. In potato, the
levels of inhibitors, including ABA, decrease during the quiescent period prior to renewed growth. ABA in extremely low concentration, moreover, prolongs the dormancy of excised potato buds.
Recently, ABA has been shown to be involved in the responses of many plants to stress conditions. As the ABA concentration increases in the leaves the stomata close. In this way, it appears that ABA is directly involved in the opening and closing of the stomata, thus regulating the rate of transpiration. Through this mechanism, ABA appears to protect the plant through conditions of water stress or drought.
At present the mechanism of action of ABA is not clearly understood. However, the available evidence indicates that ABA affects transcription as shown by reduced activity of chromatin-associated RNA polymerases. In other cases the mechanism of action of ABA appears to involve regulation of the translation of long-lived messenger-RNA, whilst its effects on stomata probably involve the regulation of membrane permeability.
13.6.2—
Role of ABA in Dormancy
One of the lines of research which led to the isolation of ABA from sycamore leaves was a change in the growth inhibitory activities in extracts of tree seedlings grown on long- or short-days. An increase in content of the growth inhibitory material in leaves was noted during the late summer and early autumn (Phillips & Wareing, 1958). Furthermore, the idea that bud dormancy in potato tubers may be caused by growth inhibiting substances had been suggested by Hemberg (1949). The original correlation of inhibitory material in leaves grown under short- and long-days and the induction of dormancy were based on measurements obtained by bioassay techniques. Recently, Lenton et al. (1973) have attempted to repeat these determinations using gas chromatography to compare the amounts of ABA present. They found that transferring birch, red maple or sycamore plants to short-days had no effect on ABA content. The importance of a balance between growth promoters and growth inhibitors has been stressed frequently. Tinklin and Schwabe (1970) have found considerably more inhibitor in bud scales than in the bud axis of blackcurrant. In these experiments the ABA concentrations were correlated with the degree of dormancy and the levels were reduced by treatments which encouraged bud break.
The most convincing evidence suggesting that ABA induces dormancy is the production of turions in Lemna polyrrhiza. When ABA is added to the medium under conditions that allow continuous growth, it not only inhibits growth but causes production of the dense, dormant, fronds known as turions (Stewart, 1969).
ABA is a potent inhibitor of seed germination, and its presence as a major growth inhibitor in dormant seeds of many species has cast it in the role of the maintainer of seed dormancy. ABA has been isolated from seeds of many genera of higher plants, and seeds of an equally large number of species have
been prevented from germination by soaking in ABA solutions. When seeds and fruit parts are separated, it is usually found that the concentration of ABA in the fruit is about 5 to 10 times greater than in the seed (Milborrow, 1974).
Lipe and Crane (1966) found that ABA in peach seeds decreased during stratification. Obviously, the part played by endogenous growth promoting compounds and the balance between them and ABA during the breakage of dormancy needs to be explored in more detail. Certainly, the balance between the two kinds of regulators is important. ABA is not an irreversible inhibitor since one of its most striking features is the facility with which it can be leached out of treated seeds thereby allowing the resumption of growth.
13.6.3—
Effects on Abscission
In the last few years several hundred experiments have been carried out in which plants, and parts of plants, of many genera have been treated in a variety of ways with ABA. It is quite unusual for any report to show clearly that the hormone controls abscission of leaves, which leads to the conclusion that exogenously applied ABA has little effect on leaf abscission. Nevertheless, ABA was first isolated by following its abscission-accelerating activity in petiolar stumps of cotton explants. The growth inhibitory action of this factor was believed to be responsible for the premature abscission of immature young lupin fruits (Cornforth et al., 1966). Consequently, the abscission-accelerating effect of ABA is well documented and extensively discussed (Milborrow, 1974).
Many of the experiments reporting leaf abscission have been carried out on tree crops using extremely high concentrations of ABA and often near the end of the growth season in attempts to cause abscission of fruits. The observed stimulation of leaf abscission may be an indirect effect of non-physiological high concentrations which stimulate ethylene production (Edgerton, 1971). For example, Cooper and Henry (1968) treated orange trees with sprays of 500 µg ml–l of ABA in summer and winter. The summer treatment caused leaves to develop colour and fall, but the winter treatments had no such effects. Olive trees were found to suffer some leaf abscission in one experiment but no effect was observed in another.
It appears, therefore, that ABA is not closely involved in the regulation of leaf abscission. The test system in which it has a stimulatory action consists of isolated petiolar explants containing presumptive abscission zones and maturing leaves nearing the end of their life. Even this tissue requires application of abnormally high concentrations of ABA to manifest an effect.
The role of ABA on fruit abscission is more certain. The early work of Van Steveninck (1959) showed that the inhibitor now known as ABA was intimately involved in the abortion of the young immature fruit of lupin. Application of ABA to mature peach, olive, apple and citrus fruits accelerated abscission and the effect of ABA was also marked on young grape flowers and berries (see Milborrow, 1966).
13.6.4—
Effects of ABA on Wilting
Wright (1969) found that when cut shoots were wilted there was an increase in the concentration of the so-called b -inhibitor. He went on to identify this inhibitor as ABA and defined the conditions under which the increase occurred. A water loss of about 10% in total fresh weight caused approximately 40-fold increase in ABA content while further water loss had no additional effect. Wright and Hiron (1969) have reported that other stress conditions, such as waterlogging, caused a similar rise in ABA, but they point out that such treatments cause wilting by reducing the efficiency of water uptake. The surprising feature of the increase in ABA is the rapidity with which it occurs. The content in turgid bean leaves has been calculated from the results of Wright and Hiron (1969). They show that ABA content increases from a normal level of 6 µg kg–1 fresh weight to 7 µg kg–1 within 7 minutes after blowing a dry and warm air stream across the plants. This level increases to 33 µg kg–1 within 25 minutes and 68 µg kg–1 within 45 minutes. In other experiments they show that in wheat leaves the ABA content increased from 23 to 171 µg kg–1 fresh weight within 4 hours of wilting. The ABA content of bean leaves remained at 67 µg kg–1 while they were kept waterlogged for 5 days. The implications of these data have yet to be explored in detail, but the observations offers a feasible explanation of the reduced growth of crops suffered during drought.
The extra ABA is probably formed by synthesis rather than by release from a precursor or conjugate because much more labelled mevalonate was incorporated into ABA by a sample of leaves that had been fed and then wilted than similar leaves which were kept moist during the entire experiment. Furthermore, the presence of 40 times the amount of a precursor or conjugate probably would have been detected by extraction and bioassay techniques. Many types of experiments have shown dramatic increases in ABA content on wilting in french beans, brussel sprouts, sugar cane, wheat, avocado spinach, cotton, peas and tomatoes (see Milborrow, 1974).
Perhaps the best information supporting a direct involvement in the direct closure of the stomata is afforded by a wilty tomato mutant produced by X-irradiation (Imber & Tal, 1970). The shoots of this plant contain one-tenth the amount of ABA contained in the normal variety. Applications of 0.1 to 10 µg of ABA caused a rapid and progressive reduction in transpiration rate of leaves and leaf discs. With 10 µg ABA ml–1 the stomata closed in darkness.
13.6.5—
Affects of ABA on Enzyme Activities
The ability of plant hormones to affect directly the activity of enzymes has not been extensively investigated. When an effect has been reported it is smaller than would be expected if the hormone specifically regulates at that site. Hemberg (1967) reported that ABA can inhibit a -amylase in vitro, but as the enzyme used was extracted from fungi the significance as related to higher
plants requires additional investigation. ABA has been shown to complex with fungal a -amylase and thereby change its physical properties. Saunders and Poulson (1968) found a slight stimulation of invertase activity at 10–7 M ABA and slight inhibition at 5 × 10–7 M and above. Again the significance is difficult to assess since a fungal enzyme was used.
13.6.6—
Effects of ABA on Nucleic Acid Synthesis
The first observations of the effects of ABA on nucleic acids were made by Van Overbeek et al., (1967) using Lemna. The incorporation of radioactive phosphate (32 P) into nucleic acid fractions was inhibited by ABA but reversed by benzyladenine. The primary site of action of ABA cannot be deduced with certainty because of the time scale and the complexity of the responses. Subsequent work has indicated that DNA synthesis is almost certainly not the primary target of ABA (Villiers, 1968). This has been clearly shown to be the case in dry wheat embryos by Chen and Osborne (1970) who found that protein synthesis commenced from imbibition and was inhibited at 6 hours by ABA, whereas RNA synthesis, as measured by the incorporation of 3 H-uridine, was not measurable until 12 hours. In the same experiments the incorporation of 3 H-thymidine into DNA was measurable after 24 hours. Experiments by Pearson and Wareing (1969) have shown that chromatin-directed RNA polymerase activity was slightly inhibited by 0.26 m g ml–l of ABA when added to the grinding medium. However, when ABA was added to the purified chromatin little or no effect was noted. Bex (1972) also reports that ABA has no effect on the binding between nucleohistones and DNA as measured by their melting point measurements. Schwartz (1971) has shown that ABA can alter the balance of alcohol dehydrogenase in maize, but the inhibitor was added during the growth of the cells. Thus, while it appears that ABA has small and random effects on nucleic acid biosynthesis in a number of plants, it is reasonably clear that the hormone has little or no direct effect on the synthesis of nucleic acids at the level of the genome.
13.6.7—
The Involvement of Abscisic Acid in Messenger-RNA Translation
Ihle and Dure (1972) investigated the appearance of various enzyme activities during the germination of cotton seeds and embryos. They showed that protease and iso-citrate lyase appear to be synthesized de novo during germination utilizing messenger-RNA which had been transcribed much earlier when only about 60% completion of embryogenesis had been reached. The translation of these messenger-RNAs during the last 40% of embryogenesis was apparently prohibited by the presence of ABA diffusing into the embryo from the ovule wall. The mode of action of ABA in maintaining translation inhibition appears to
involve RNA synthesis as judged by the fact that the ABA inhibition was inhibited by the presence of actinomycin D. Translation of the required messenger-RNAs for the germination enzymes may be induced prematurely by simply dissecting the ovules from the plant, suggesting that the breakage of the connection between the ovule and the mother plant may be required for the in vivo induction of translation. This hypothesis is further substantiated by the observation that, in vivo, the connection between the ovule and the placenta is normally severed at 60% completion of embryo-genesis.
The results coming from Dure's laboratory are the only ones at present which show that ABA may have a direct effect on the translation of messenger-RNA pre-existing in the embryo tissue.
13.6.8—
Summary
Even though ABA was first found in cotton plants and dormant sycamore leaves, it seems reasonably clear that this hormone has little or no effect on either the abscission of leaves or the dormancy of buds. It appears that the most likely physiological action of ABA on plants is to control the abscission zone in the fruit pedicel and thereby allow abscission to take place and cause fruit drop. Secondly, ABA very definitely appears to be involved in stomatal closure in response to stress conditions, particularly drought. In terms of a more biochemical approach it appears that ABA has little effect on various enzyme activities. Those enzymes studied show only small enhancement, or in some cases, inhibition, by ABA. Generally it has been shown that ABA inhibits the synthesis of various nucleic acids.
Again, one must be drawn to the conclusion that ABA has little effect directly on nucleic acid synthesis at the genome level. The most excitingp aproach to abscisic acid action appears to be that found in the cotton embryo where it has been shown to inhibit the translation of pre-existing messenger-RNAs found in the developing embryos. Even this response probably involves more complex processes than a direct inhibitory effect, since actinomycin D inhibits the ABA-induced inhibition. These data possibly imply that a suppressor molecule has to be formed to bring about effects.
Further Reading
Abeles F.B. (1973). Ethylene in Plant Biology. Academic Press, N.Y. 302 pp.
Cherry J.H. (1973). Molecular Biology of Plants, A Text-Manual. Columbia University Press. N.Y. 204 pp.
Hall R.H. (1973). Cytokinins as a probe of developmental processes. Ann. Rev. Plant Physiol.24, 415–44.
Jones R.L. (1973). Gibberellins: their physiological role. Ann. Rev. Plant Physiol.24, 571–98.
Key J.L. (1969). Hormones and nucleic acid metabolism. Ann. Rev. Plant Physiol.20, 449–74.
Milborrow B.V. (1974). The chemistry and physiology of abscisic acid. Ann. Rev. Plant Physiol. 25, 259–307.
Runeckles V.C., Sondheimer E. & Walton D.C. (eds) (1974). The Chemistry and Biochemistry of Plant Hormones. Vol. 7. Recent Advances in Phytochemistry. Academic Press. N.Y. 178 pp.
Skoog F. & Armstrong D.J. (1970). Cytokinins. Ann. Rev. Plant Physiol.24, 359–84.
Varner J.E. & Johri M.M. (1968). Hormonal control of enzyme synthesis. In Biochemistry and Physiology of Plant Growth Substances (eds. F. Wrightman & G. Setterfield). The Runge Press Ltd. Ottawa. pp. 793–814.