Hydrovolcanism
Hydrovolcanism is a broad term that encompasses the role of external (nonmagmatic) water in volcanic activity; synonyms include phreatomagmatism and hydromagmatism . This topic may have its roots in the 18th Century Neptunists' theory about the origin of basaltic rocks in oceans (which was later formalized by Abraham Werner). After the eruption of Krakatau in 1883, world attention was focused on the dynamic potential of oceanic volcanism (Verbeek, 1885). Because water plays such a fundamental role in geothermal systems, we will briefly describe some research efforts that have unraveled the complexities of water/magma interactions in volcanic settings. This research has led to the development of systematics for inferring the existence of external water in volcanic areas. Such systematics concentrate on the interpretation of volcanic landforms and tephra deposits, which is viewed as a first step toward finding areas in which both a heat source and water exist. The study and characterization of hydrovolcanic features is chiefly used to make quick estimates of the abundance of water in a hydrothermal system. Detailed studies of water/magma interaction constrain subsurface conditions that have evolved within a geothermal system; for example, depth and lithology of aquifers and permeable formations, temperature of hydrothermal alteration, and spatial and temporal variations in subsurface hydrothermal behavior.
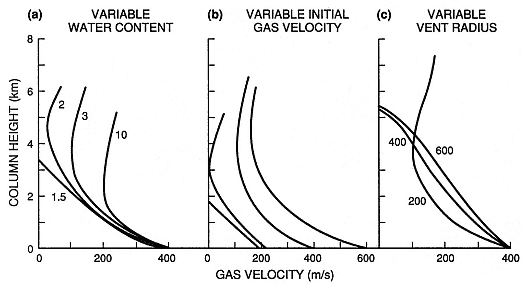
Fig. 1.15
Velocity/height profiles for the lower part of a Plinian eruption column. Calculated by the method
proposed by Wilson (1976), the plots show consecutively the effect of (a) variable water content
(n = 1.5, 2, 3, and 10 wt%), with a constant initial velocity of 400 m/s, and a constant vent radius
(rv ) of 200 m, (b) variable initial velocity with n = 3.0 wt% and rv = 200 m, and (c) variable vent
radius (rv = 200, 400, and 600 m) with constant initial velocity and water content (n = 3.0 wt%).
(Adapted from Sparks et al ., 1978.)
A host of natural phenomena are produced by the interaction of magma or magmatic heat with an external source of water. Because the earth's crust is, in general, saturated with water, most volcanic fields have at least one feature produced by hydrovolcanic phenomena. Most widely recognized are phreatomagmatic and phreatic explosions (see Appendix G for definitions). Many hydrovolcanic phenomena, such as the gradual fracturing of country rock around magma intrusions and the alteration of rocks in hydrothermal systems are neither explosive nor readily observable. In their review of hydrovolcanism, Sheridan and Wohletz (1983a) discuss various aspects of research, including
· geologic environments where systems occur,
· the range of physical phenomena,
· the wide variety of classical eruption types and landforms,
· experimental modeling,
· petrography of hydrovolcanic products,
· textural analysis and indicators of water abundance in deposits, and
· hydrovolcanic cycles.
During recent years, hydrovolcanism has developed as a field theory that applies to a range of physical as well as chemical processes (for example, magma differentiation by fluid and vapor transport, dynamic magma alteration during eruption, and contamination of magma bodies by external water). The brief review included in this chapter serves as an introduction to more detailed considerations of hydrovolcanism and geothermal energy that are provided in later chapters.
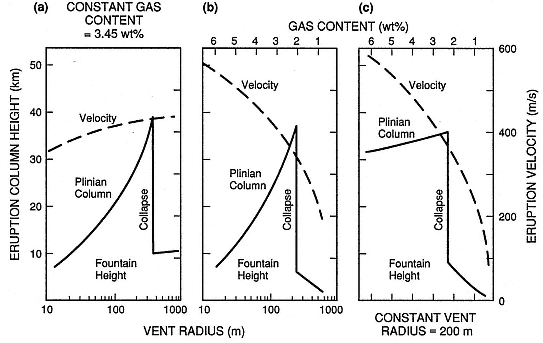
Fig. 1.16
Prediction of column collapse as a function of (a) increasing vent radius, (b) decreasing exsolved
gas content and increasing vent radius, and (c) decreasing gas content with constant vent radius.
These scenarios can be used to interpret emplacement of Plinian falls and pyroclastic flows
under changing eruption conditions.
(Adapted from Wilson et al ., 1980.)
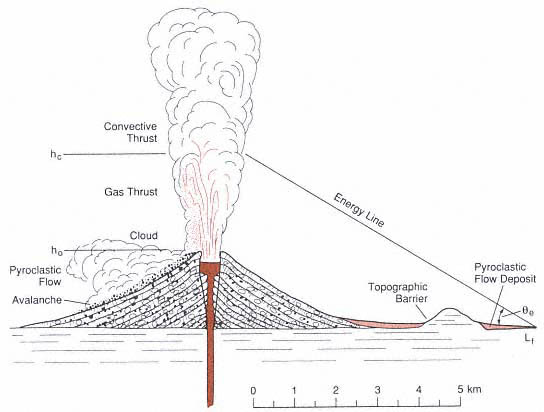
Fig. 1.17
Illustration of the "energy-line" concept for a Plinian eruption from a hypothetical composite cone.
The gas and convective thrust regions of the eruption column (Wilson, 1976; Sparks et al ., 1978)
are shown with a pyroclastic flow that is initiated by collapse near the top of the gas thrust
region (hc ) with the vent at a height of h(0). The initial potential velocity of the pyroclastic flow is
constrained by v(0) = [2gD h(0)]1/2 , where D h(0) = hc - ho . Flow acceleration a(i) and runout are
a function of the local topographic slope [q (i)] and the Heim coefficient (µh ) shown by Eq. (1-17).
The general slope of the energy line (qe ) is given by arctan (hc /Lf ) for which Lf = the distance from
the vent where v(i) vanishes (that is, where the energy line intersects the topographic surface).
(Adapted from Sheridan, 1979.)
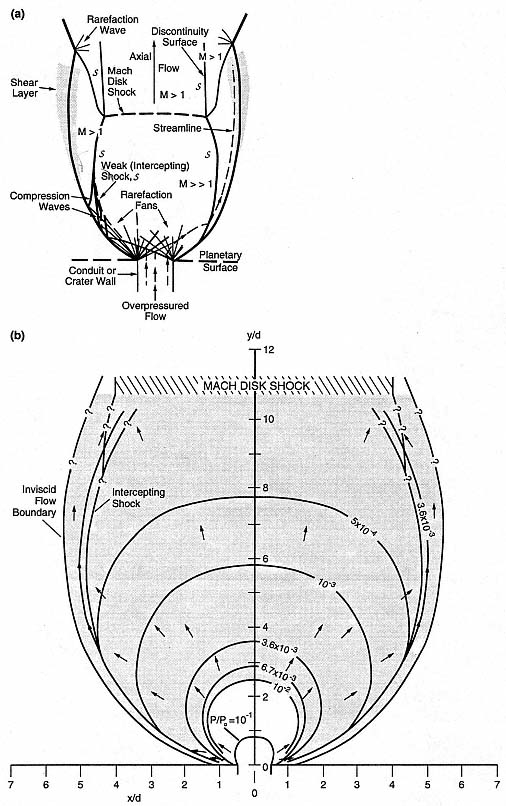
Fig. 1.18
Schematic of (a) an idealized overpressured jet and (b) the jet structure calculated for
conditions of supersonic flow [overpressure of 12.5 MPa; Mach number (M) = 1.02] as
they occurred during the lateral blast and pyroclastic surge of Mount St. Helens on
May 18, 1980. Dimensions x and y are scaled to vent diameter (d @ 0.5 km).
An overpressured jet rapidly expands through rarefaction waves [lines that extend from
the conduit vent sides in (a)] that form a rarefaction fan. As the fluid expands, its atmospheric
boundary initially diverges from axial flow, giving the jet a flaring structure. With substantial
overpressure, the jet may overexpand, developing a subatmospheric pressure zone in the
center of the flow [shaded zone in (b)], which allows the atmosphere to cause downstream
constriction of the jet. Reflections of rarefaction waves from the atmospheric boundaries of
the jet form compression waves that coalesce into weak (intercepting) shocks, which in turn
coalesce downstream to set up a Mach disk shock. Zones of supersonic and subsonic flow are
designated by Mach number. Across the intercepting shocks, the flow velocities decrease,
whereas pressure increases and streamlines (dashed lines with arrows) are deflected.
The supersonic area of the jet, upstream from the Mach disk, corresponds approximately to
the zone of directed blast devastation and pyroclastic surge deposits.
Environments of Hydrovolcanism
During its ascent to the surface, magma commonly encounters groundwater; connate water; marine, fluvial, or lacustrine water; ice; or rain water. The subaqueous environment includes all activity beneath a standing body of water (Kokelaar, 1986); products of this activity have been called subaquatic (Sigvaldason, 1968), aquagene (Carlisle, 1963), hyaloclastite (for deep marine; Bonatti, 1976), hyalotuff (for shallow marine; Honnorez and Kirst, 1975), and littoral (Wentworth, 1938). Volcanism that heats groundwater to produce steam explosions that do not eject juvenile magma fragments is called phreatic (Ollier, 1974) or hydrothermal (Muffler et al ., 1971; Nairn and Solia, 1980). Subglacial volcanism (Noe-Nygaard, 1940) is noted by its products, including massive floods (jökullaups ), table mountains (stapi ), and ridges (mobergs ).
The wide variety of hydrovolcanic phenomena underscores the fact that interaction between water and magma or magmatic heat should be expected in any volcanic setting. One long-held theory suggests that the depth below surface at which dynamic, water/magma interaction is possible is limited by the critical pressure of water or water-rich fluids, and that above this pressure, the phase change from liquid to gas upon heating does not involve large-volume changes (Lorenz, 1986). Accordingly, depths of 0.8 to 2.2 km were considered limits to explosive magma/water interaction. However, more recent work suggests that the critical point need not be a limitation to dynamic interaction and that expansion of water through its two-phase field is not required for rapid volume changes (Wohletz, 1986).
Nature of Hydrovolcanic Phenomena
The physical phenomena of hydrovolcanism belong to a class of well-studied physical processes termed fuel-coolant interactions (FCI). Fig. 1.20 depicts a hypothetical geologic
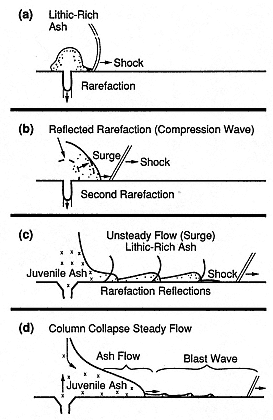
Fig. 1.19
illustration of the calculated pyroclastic surge
generated during initial moments of Plinian
eruption. (a)An overpressured burst propagates
a bow shock into the atmosphere ahead of
lithic-rich ash as a rarefaction wave recedes
into the magma reservoir. (b and c) Reflected
rarefactions from the reservoir and flow margins
form weak shocks that accelerate ash in surges.
Together, these phenomena constitute a blast
wave that precedes (d) the flow of
juvenile ash from the vent.
(Adapted from Wohletz et al ., 1984).
system in which magma (fuel) explosively interacts with water-saturated sediments (coolant). This process occurs in stages of (a) initial contact and steam-film development, (b) coarse mixing of magma and water or water-rich rock, (c) vapor expansion and flow, and finally (d) explosion and fine fragmentation of the magma. The process does not necessarily evolve through all these stages and may be arrested, for instance, before mixing or explosion.
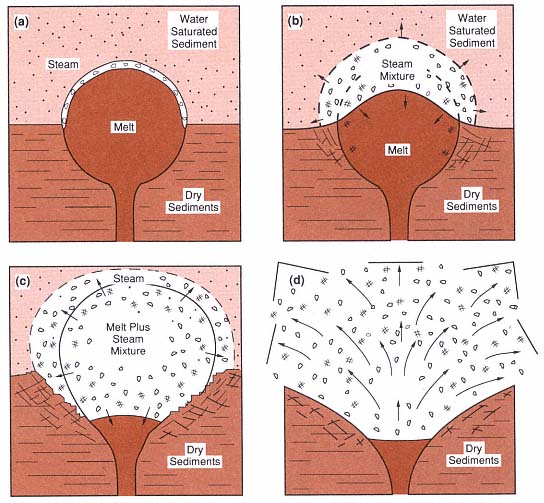
Fig. 1.20
Hypothetical setting of subsurface hydrovolcanic activity, showing (a) initial contact of magma with
water-saturated sediments, (b) vapor film growth, (c) mixing of magma with the sediments, and
(d) expansion of the high-pressure steam in an explosion.
(Adapted from Sheridan and Wohletz, 1983a.)
Much of our theoretical-understanding of hydrovolcanism has developed from laboratory experiments (for example, Wohletz and McQueen, 1984). This approach has made it possible to quantify some controlling parameters by using field and laboratory measurements of hydrovolcanic products. Figure 1.21 shows results from early experiments (Sheridan and Wohletz, 1983a), in which the mass ratio of water and magma (thermite analog) interacted and confining pressure controlled the explosive efficiency of the system.
The thermodynamics of heat transfer is also a significant aspect of hydrovolcanic systems and their physical and chemical effects. The mechanical work produced by interaction of magma with external water is partitioned into many possible modes, including fragmentation of the magma and country rock; excavation of a crater; dispersal of tephra; seismic and acoustic perturbations; and chemical processes such as solution and precipitation, mass diffusion, and magma quenching and crystallization.
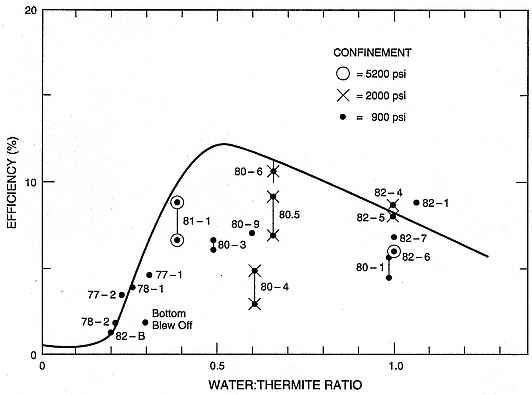
Fig. 1.21
Some results of experimental studies (indicated by number) by Wohletz and McQueen (1984)
showing explosive efficiency as a function of water-to-magma mass ratio and confining pressure.
Note the apparent maximum near 0.5 and the increased explosivity of high-confinement
pressure tests. Explosive interaction occurred at 5200 psi (~350 bars) confining pressure,
which is well above the previously assumed limit at water's critical pressure.
This work, D Wsys , is the sum of changes in kinetic energy (D Ek ), potential energy (D Ep ), and volume-change work (pD Vsys ), which is given by

There are several ways by which to evaluate the above expression, but one of the most direct methods is to estimate the change in the internal energy of the water/magma mixture (D Umix ); by definition D Wsysº -D Umix , where

and m = mass, U = internal energy, and subscripts w and m denote water and magma, respectively. Wohletz (1986) demonstrated a method for evaluating Eq. (1-23) that requires data from extended steam tables (Burnham et al ., 1969; Haar et al ., 1984). Further consideration of the mixing and explosion stage (Wohletz, 1986) yields information on particle velocities and sizes. Heiken et al . (1988) extended these calculations to explain country rock fracture by hydrovolcanic processes.
Experimental investigations of water/magma interactions have displayed a variety of explosive and nonexplosive behaviors that are analogous to natural volcanic activity. These results support observations of hydrovolcanic eruptions in which a wide variety of classical eruption types (for example, Strombolian,
Surtseyan, Vulcanian, and Plinian) have involved external water. There is additional evidence of hydrovolcanism in a variety of landforms that range from small maar/tuffring craters to some large caldera outflow sheets of tephra. Such features as peperites, mud volcanoes, hydrothermal explosion pits, pillow lavas and breccias, and parts of composite cones can also be attributed to hydrovolcanic activity. These eruptive behaviors and resulting landforms are, in a general fashion, related to the degree of water interaction, as is shown in Fig. 1.22.
Hydrovolcanic Products
Hydrovolcanic solid products are generally fragmental and are termed hydroclasts by Fisher and Schmincke (1984), instead of pyroclasts , which refers solely to the fragmental products of magmatic eruption. Hydrovolcanic solid products include tephra, explosion breccia, pillow lava, palagonitic and zeolitic tuff, lahars, blocks and bombs, silica sinter and travertine, and intrusive breccia and tuff. Some of these materials involve posteruptive processes (for example, hydrothermal) in which water interacts with volcanic products.
Petrographic studies of hydrovolcanic products involve determining the grain-size and textures of tephra and the chemical signatures caused by rapid and slow alteration. These data are indicators of the degree and type of water interaction. For example, the grain size of hydroclasts is a function of the mass ratio of interacting water and magma; grain textures are indicative of the type of interaction—passive, explosive, extensive, or transient. Field characterization of hydroclastic products focuses on (a) analysis of various ejecta deposit characteristics, including textural analysis of bedforms, lithification, and deposit thickness vs distance from the vent, and (b) correlation of these observations with vent type (for example, composite cone, tuff ring, or caldera).
A correlation can be made between the median grain diameters of hydrovolcanic products and the water/magma mass ratio (Fig. 1.23); this correlation was developed from both experimental and field applications. In general, hydrovolcanic tephra are distinguishable from magmatic tephra by their much finer grain size. Microscopic examination of grain shapes and textures also reveals hydrovolcanic features (Fig. 1.24). Quantitative analyses of these features can document the relative importance of hydrovolcanic (wet) and magmatic (dry) mechanisms in samples from deposits of mixed origins. Hydrovolcanic grain textures are also indicative of the type of water/magma interaction (for example, wet vs dry; Wohletz, 1983).
Hydrovolcanic Cycles and Geothermal Energy
Hydrovolcanic phenomena occur in regular patterns at some volcanoes and thus can assist in defining cycles that in turn are useful in both predictions of future activity and estimates of subsurface hydrological conditions. The eruptive cycles portrayed in Fig. 1.25, for example, show the changing availability of groundwater during periods of activity at several volcanoes. Cycles can be documented by careful field and laboratory analyses of volcanic products in which the abundance of erupted steam and its temperature are constrained by textural indicators of grain cohesion, deposit mobility as a function of moisture abundance, and degree of clast alteration. Cycles are characterized as "wet" when the volcanic products indicate an increase of water during the eruptions; "dry" cycles produce tephra that indicate decreasing water abundance throughout the eruption. The nature of these water indicators also demonstrates whether the erupted steam is saturated (wet) or super-heated (dry). As a general rule, locations that show wet cycles might be better candidates for geothermal exploration because
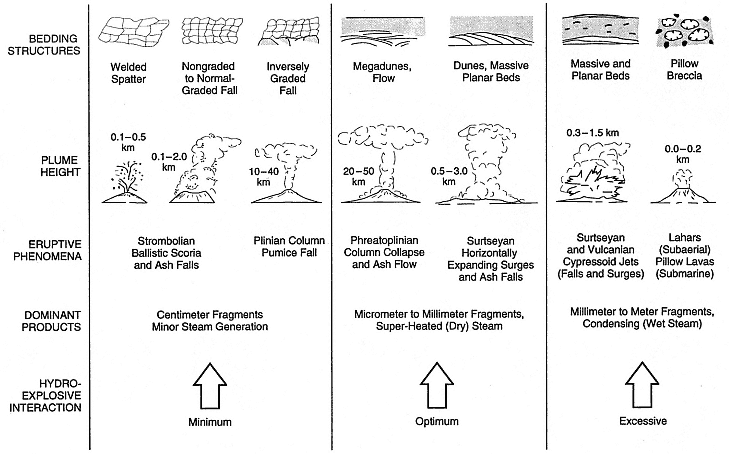
Fig. 1.22
Relationship of eruptive phenomena, deposit type, and landform to water-to-magma interaction ratio.
(Adapted from Sheridan and Wohletz, 1983a.)
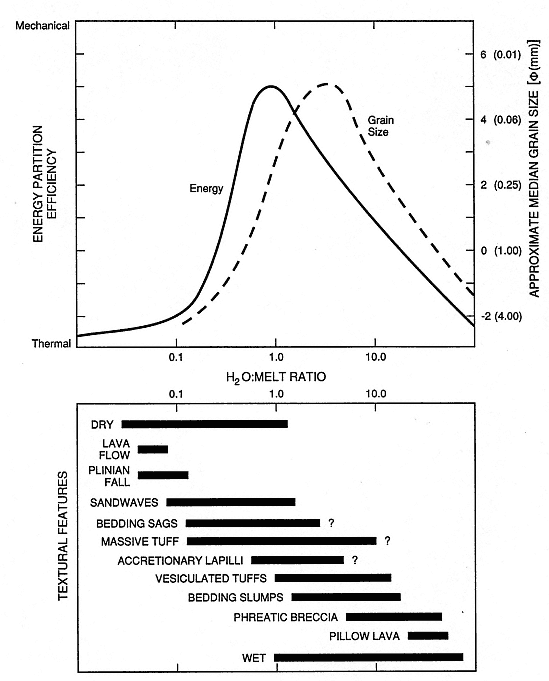
Fig. 1.23
Correlation of deposit texture and grain size to water-to-magma ratio.
(Adapted from Frazzetta et al., 1983 and Sheridan and Wohletz, 1983a.)
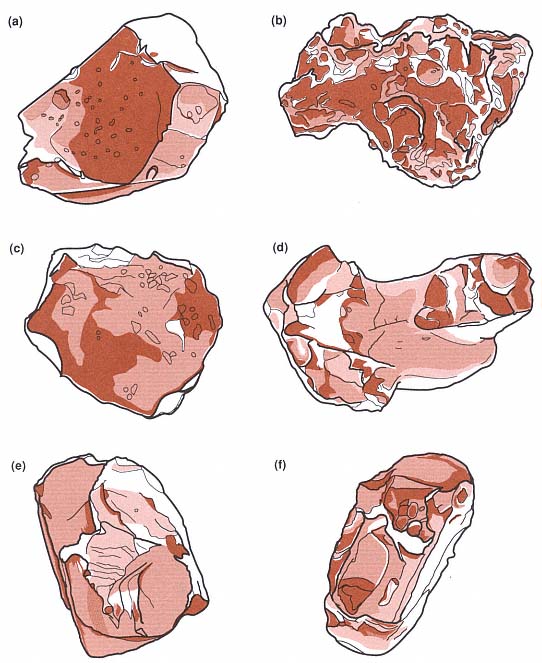
Fig. 1.24
Sketches of pyroclast textures resulting from hydrovolcanism. These textures include
(a) a characteristic blocky and equant glass shard, (b) a vesicular grain shard with cleaved vesicle
surfaces, (c) a platy shard, (d) a drop-like or fused shard, (e) a blocky crystal with conchoidal fracture
surfaces, and (f) a perfect crystal with layer of vesicular glass.
(Adapted from Sheridan and Wohletz, 1983a.)
they prove that water is sufficiently abundant in the volcanic system to quench the magma to water-vaporization temperatures. When estimating the volume of erupted hydroclastic products, this general rule constrains the volume of water involved in the eruptions and thus provides a measure of water abundance in the volcanic system.
Funiciello et al . (1976) pointed out the correlation between geothermal localities and phreatomagmatic volcanoes in Italy, especially those showing wet cycles. In addition, these authors demonstrated how the study of phreatomagmatic products helps locate and characterize a geothermal reservoir with respect to its lithology and fracture permeability, topics that Heiken et al . (1988) discussed in further detail. These studies provide an excellent background for our discussion of hydrovolcanism in Chapter 2.
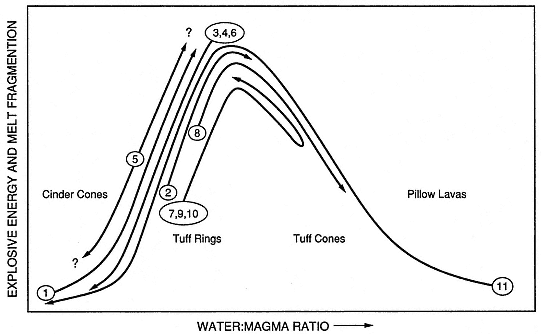
Fig. 1.25
Various cycles of hydrovolcanism displayed by several type of studied volcanoes. Temporal
variations of water-to-magma mass ratios are shown for (1) Crater Elegante, Mexico;
(2) Kilbourne Hole, New Mexico; (3) Peridot Mesa, Arizona; (4) Taal volcano, Philippines;
(5) Ubehebe crater, California; (6) Zuni Salt Lake, New Mexico; (7) Cerro Colorado, Mexico;
(8) Diamond Head, Hawaii; (9) Koko Crater, Hawaii; (10) Pavant Butte, Utah; and (11) Surtsey,
Iceland. These cycles illustrate general trends (see Sheridan and Wohletz, 1983a, Fig. 5), including
wet to dry (well demonstrated by Vulcano in the Aeolian Islands, Italy) and dry to wet
(activity characteristic of Vesuvius). Some volcanoes show reversals in cyclic activity
(7, 9, and 10 here are tuff cone structures), whereas repeated cycles between dry (Strombolian)
and wet (Surtseyan) occur at others (5).
(Adapted from Wohletz and Sheridan, 1983a.)