Introduction
The activities, potentialities, and developmental fate of all cells are determined by the hereditary genetic material stored within the nucleus, and also to a lesser but still important extent, by that stored within the plastids and mitochondria. Hereditary information is coded within the nucleotide sequences of the DNA, and the generalized concept of the expression of genetic information is usually portrayed as follows:

This 'central dogma' states that the information contained within the DNA is only expressed, in terms of cellular processes, through the action of the ultimate gene products, the proteins, most of which are enzymatic in nature. The central dogma also points to there being two principal points at which gene expression may be regulated; i.e. gene transcription and m-RNA translation. In fact, a further control point should be added, since the catalytic activity of enzymes may be regulated in several ways in addition to the regulation of the synthesis of the enzyme. Thus the regulation of gene expression may be more adequately described by the following scheme:

in which the potential control points at transcription, translation and enzyme activation/inactivation are numbered.
Section II considers the biochemical and structural basis of gene expression and its regulation in higher plants and places it in context with what is known from the more extensive studies carried out with other organisms. The principal hypothesis of the regulation of gene expression stems from the discoveries relating to the control of enzyme levels in bacteria. In 1961, to account for the known effects of metabolites on the synthesis of specific enzymes in bacteria, Jacob and Monod proposed that the activity of the genes which code for specific enzyme proteins (structural genes ) is controlled by other genes, not necessarily linked to the structural genes, and known as regulator genes. A regulator gene codes for the synthesis of a specific cytoplasmic protein, known as a repressor, which has the capacity to interact with a region of chromosomal DNA (the operator ) adjacent to the initiation end of the structural gene. The binding of a repressor to a specific operator, which may control a sequence of structural genes (known collectively with the operator as an operon ) inhibits transcription of m-RNA on those structural genes. It is also held that the repressor protein has the capacity to bind one or more low molecular weight
substances, known as effectors, which may alter its capacity to bind to the operator. Effectors which combine with the repressor and prevent its binding to the operator are termed inducers, since they allow enzyme synthesis from a previous repressed state; inducers are commonly substrates of the first enzyme in a pathway. Effectors which combine with the repressor and enhance binding with the operator, inhibit or repress enzyme synthesis; such effectors are known as co-repressors and are commonly products of a reaction pathway. In repressible systems of this nature the structural genes are normally fully expressed in the absence of the co-repressor and the enzymes are thus known as constitutive. As shown in Fig. 11.1 this hypothesis elegantly accounts for the formation of enzyme in response to the substrate (enzyme induction ) and for the cessation of enzyme formation in response to the end product (enzyme repression ).
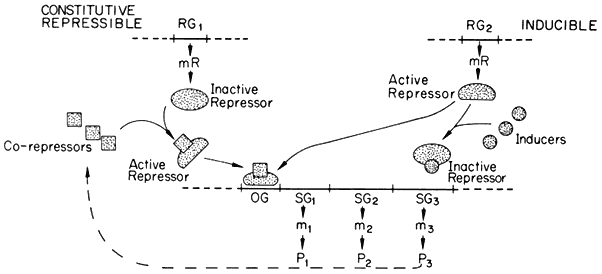
Figure 11.1
A generalized scheme of the Jacob–Monod model for constitutive repressible
and inducible systems of gene regulation. The regulator genes (RG) are shown as
coding for the formation of either active repressors (Inducible system) or inactive
repressors (Constitutive repressible system) which bind to the operator gene (OG)
and block the transcription of the structural genes of that operon (SG1 to SG3 ). P =
protein and m = mRNA; P3 is considered to be an enzyme whose catalytic activity
leads to the formation of a co-repressor for the constitutive repressible system.
(Modified with permission from F.A.L. Clowes and B.E. Juniper
(1968) Plant Cells. Blackwell Scientific Publications Ltd., Oxford.)
It is not yet clear how fully these concepts account for gene expression and its regulation in eucaryotic organisms. As will be seen from Chapter 9, the structure, organization and complexity of nuclear DNA are wholly different from the rather simple arrangements found in bacteria. There seems to be far too much DNA than would be necessary to code for the predicted number of structural genes and attendant regulator genes as estimated from the Jacob-Monod model. Several suggestions have been put forward to account for this unexpected genetic largesse, but the most likely ideas involve the extra DNA in the regulation of the structural genes (see Britten & Davidson, 1969).
Additionally, the separation of the site of transcription (in the nucleus) from that of translation (in the cytoplasm) creates further opportunities for regulation. It will be seen that the processes of RNA and protein synthesis in the nuclei and cytoplasm of plants are similar in principle, but different in detail, from those in higher animals (chapters 9 and 10).
A very important aspect of plant molecular biology, and one which is unique to plants, is the presence of a substantial store of genetic information in the chloroplasts (chapter 11). The coding potential of chloroplast DNA is vastly greater than that of mitochondrial DNA, and many of the activities of higher plant cells, and indeed a large part of their total protein, is coded for in the chloroplast genome. The regulatory processes involved here must be highly complex, since it is known that some chloroplast proteins are coded for and synthesized within the plastids, and others coded in the nucleus, and synthesized in the cytoplasm; Fraction I protein, the most abundant protein in green leaves, is composed of two types of sub-units, one of nuclear, and one of plastid, origin. Clearly, here the regulation of gene expression must involve the integration of transcription and translation within three separate cell compartments, the nucleus, the cytoplasm and the chloroplast.
As stated briefly above, gene expression may also be regulated after the final gene product, the protein, has been formed, since a gene can only be considered to be expressed when the protein it codes for performs its specific function. Thus the activation and inactivation of enzymes, through the several known mechanisms, becomes properly a facet of gene expression (chapter 12).
The integration of the activities of different cells, particularly with regard to development, inevitably requires on the one hand, the generation and transmission of intra- and inter-cellular stimuli and, on the other, specific responses to these stimuli. In higher animals, both electrical and chemical stimuli appear to be important; in higher plants inter-cellular integration seems to be almost completely chemical, through a narrow range of hormonal substances. Although understanding of the mechanism of hormone action in plants is still incomplete, the regulation of gene expression is probably involved (chapter 13).
All organisms need to be able to perceive their environment and adapt to environmental changes. Evolution, having favoured non-motility for plants, has inevitably endowed them with extremely subtle mechanisms for detecting and responding to certain environmental factors. The best example here is the response of plants to light, in which specific photoreceptors react to signals from the radiation environment by initiating changes in metabolism and development. As an example of external regulation of gene expression in higher plants, chapter 14 deals with the mechanism of action of phytochrome, an important photoreceptor found in all green plants.
Section II therefore, is concerned with the genetic material of higher plants, its organization, complexity and regulation, with particular reference to the control of cell metabolism, to cell-cell communication in hormone action and to responses to the environment via photoreceptor action.