XVII. Animals in Relation to Physical-Chemical Properties of the Environment
ECOLOGICAL GROUPS AND SOME OF THEIR ADJUSTMENTS AND CONDITIONS OF LIFE
The study of world distribution of marine animals is the function of marine zoogeography. Its chief aim is the characterization of the animal world of the sea. In causal zoogeography we seek the causes that are or have been operative to bring about the type of animal distribution actually found in present-day faunas, whether benthos, nekton, or plankton.
In the earliest studies attempts were made to establish arbitrary zoogeographic boundaries along lines of latitude, and so forth, but since these have no ecological significance rational faunal boundaries could not result, and it was not until boundaries were drawn to follow certain isotherms that the faunal divisions were also more or less clearly circumscribed. Even this does not necessarily result in rational zoogeographic boundaries because, after all, it is the actual distribution of animals themselves that forms the basis for zoogeographic divisions, since the thermal relations or other factors are not always clear. However, animal distribution (as well as other biological phenomena in the sea) is not haphazard but has resulted from orderly events some of which, although historical, may have lost their continuity, while others are continually operative as single or multiple factors conditioning dispersal, survival, and abundance. Any apparent absence of order results from lack of sufficient information with which to decipher and trace the complicated patterns that result from the inherent nature of the organisms and the factors in the environment. Marine ecology, which is concerned with the organisms in relation to their present-day environmental conditions, is a vital link in the study of zoogeography and many other biological problems of the sea.
The ecological groups to be considered in this division of the present chapter are (a) benthos, the animals of the sea floor; (b) nekton, the swimming animals; (c) zooplankton, the floating animals.
Benthos, Animals of the Sea Floor
Wherever successful dredging operations have been conducted, the ocean floors have been found to be inhabited by benthic animals from the Arctic to the Antarctic and from shore to the greatest depth. However, the number of animals (that is, the concentration per unit area) varies greatly; moreover, the kinds of animals that make up the major portion of the population differ, especially the species, genera and families. Such differences are apparent in the populations of such small topographic units as biotopes, as well as in the larger environmental divisions, and they are the biological criteria for establishing the vertical zones, littoral, archibenthic, and abyssal-benthic, as well as the horizontal faunal areas to be discussed later. It is mainly with the fauna of these larger divisions that we shall deal. For greater detail on the zoogeography of the seas the reader is referred especially to Ekman's text (1935), in which an extensive bibliography is also included.
Animals of the Littoral Zone. The outstanding feature of the littoral zone, especially of the upper or eulittoral zone extending to depths of about 40 to 60 m, is the great diversity and variability of the physical-chemical conditions of habitats. The substratum varies from clean firm rocks to shifting sands and soft muds. Marked salinity gradients sometimes exist, and seasonal and diurnal fluctuations add variety to the life of animals of this zone. Wave and tide actions are highly important, particularly in the shallower portions. Morphologically, the animals are variously modified along special lines associated, for instance, with the type of bottom, degree of exposure, depth, feeding habits. Many of the sessile forms, such as the limpets and chitons of the intertidal zones, are flattened and streamlined the better to withstand the wash and impact of rushing waters. Mussels are securely attached by strong and flexible byssus threads, while adult barnacles, corals, tube worms, and encrusting Bryozoa are rigidly and permanently cemented to rocks, shells, or other solid objects. Less rigidly attached are the hydroids, sponges, and anemones. The sessile or immobile habit so conspicuous in vast numbers of adult marine organisms is highly characteristic of life in the sea. This mode of life is made possible only by the continuous supply of floating microscopic food and the water movements necessary for its production and dispersal (see following chapters).
Among the free-moving bottom forms we find adaptations and habits so varied that all conceivable habitat facies are used. The sea urchin, Strongylocentrotus purpuratus, for example, is able to bore into rocks for protection on exposed coasts. The shells of molluscs are frequently of more sturdy structure when grown on exposed, wave-washed shores. Corals on exposed reefs are massive and compact in comparison to the more fragile and branched types found in lagoons (Vaughan, 1919).
On muddy or soft bottom environments other adaptive modifications result. The shelled animals of these environments build relatively thin, fragile shells as compared with those of animals in exposed or rocky situations. Burrowing bivalves of muddy, sandy bottoms commonly possess an enlarged “foot” useful in digging, and the siphons are elongated to extend above the substratum for intake of water providing food and oxygen. In contrast, the bivalves of hard bottoms may have these structures much reduced, and in more active forms like the scallops, tactile organs and even eyes are developed on the mantle edge. Creeping snails possess a broad foot to aid in gliding over soft mud. Burrowing worms are able to maintain permanent or temporary tubes by means of a mucous or fibrous lining secreted by the animals. Many mud-inhabiting animals are detritus feeders, eating the mud for the organic material it contains or sucking up the detritus that has settled at the mud-and-water interface.
In the littoral zone there is an abundant supply of food for animals. This results directly from favorable conditions for the production of plants, both attached and floating, and from the availability of these plants directly or indirectly to the benthic animals. An appreciable amount of organic material of terrigenous or fresh-water origin must supplement the great quantities produced in this zone. Due to this ready supply of food, the littoral zone produces benthic animals in abundance. The actual concentration is variable, of course, depending upon such local conditions as type of bottom, rate of flow of overlying water, river outflow, and upon meteorological conditions. The last is especially pronounced in intertidal situations, where seasonal rains and freshets may dilute tide pools and exposed flats with devastating results to the more sensitive species. Unusual temperatures during exposure on the foreshore result in great losses (p. 844). Most animals of this zone have a wide range of tolerance to changing conditions, but the selective action of the environment in certain areas may produce a great concentration of the species most suited to the conditions. For example,
Most littoral areas are provided with good circulation, owing to irregular bottom configuration, to the effect of tidal actions and winds, and to seasonal or diurnal convection. However, in some bays the exchange of waters may be sluggish, with the result that the free oxygen is used up by decomposing organic matter—usually abundant in such bays—and that hydrogen sulphide is produced, making for precarious if not fatal living conditions. Extreme cases of this nature are found in certain threshold fjords of Norway, where the mouth of the fjord is partially cut off from the sea by a sill of shallower depth than the inner portion of the fjord and where fresh water from adjacent land drainage may form a thin top layer. These situations offer an excellent example of the effect of physical-chemical circumstances on the success of animal life. Normally in these pools there can be no exchange of water between the ocean and the deeper water of the fjord below the sill depth because the water flowing over the sill is of lower density than the deep water of the fjord. Much of the organic material resulting from the plankton and its dependent life in the upper layers sinks to the bottom and, in decomposing, depletes the oxygen supply of the bottom water. Highly toxic conditions, resulting particularly from production of hydrogen sulphide, make it impossible for benthic aerobic life to inhabit the bottoms of these fjords. In some fjords oysters are cultivated, but they must be kept suspended in racks above the hydrogen-sulphide-charged bottom water. The layer of fresh water insulates the lower water and may make for tropical submarine climate with temperatures rising to 30°C (p. 871). Occasionally unusual circumstances, such as continuous offshore winds, build up an offshore gradient that forces upward the heavier deeper oceanic water outside the sill to a height sufficient for it to flow over the sill into the pool, where it lifts the lighter toxic hydrogen-sulphide-laden waters toward the surface. This water is lethal to the fjord animals, fishes, and invertebrates, which upon death sink to the already organically rich muds of the bottom. These or similar catastrophic circumstances lead to mass fossilization of littoral marine life; and,
Horizontal Distribution of the Littoral Fauna. As a result of faunal studies and compilation of the work of many specialists, Ekman in his Tiergeographie des Meeres (1935) has divided the seas into faunal zones characterized by the species, genera, and families of animals found within the littoral zone of these regions (fig. 220). The animals thus employed include not only the littoral benthos but also pelagic forms that are bound by their life histories to the coastal zones. In such an analysis, the assemblage of species and genera of different animal groups that are confined to or are characteristic of the population of an area is the criterion for establishing the faunal regions. Obviously the number of endemic genera or of higher taxonomic orders is often of greater significance than the number of endemic species in distinguishing a faunal region, for the species are biologically of more recent origin. For example, the Pacific and the Atlantic tropical faunas of America, though now separated by the isthmus of Panama and having only relatively few species in common, show by numerous common genera (33 for certain crabs) and by geminate (closely related) species that these faunas were a continuous fauna in past geologic ages when the two great oceans were connected in this tropical region.
The faunal areas are not sharply defined, of course, and the boundaries are to be considered transition zones the width of which is determined largely by hydrographic features, water temperature being a cardinal determining factor. But other factors also determine the geographical extent of a faunal area. These are especially continental land barriers or broad expanses of deep water such as the East Pacific oceanic barrier (fig. 220). This, owing to its depth, precludes spreading of adult littoral forms through the abyssal zone, and, because of the vast horizontal extent of water between the American shores and the easternmost Polynesian Islands, prevents transport of pelagic larval stages of littoral forms except when these stages are of specially long duration.
Broadly speaking, the littoral fauna may be divided clearly into arctic, tropical, and antarctic. Between these there are gradations giving rise to such divisions as boreal, temperate, or antiboreal Kerguelen fauna. Some of the faunal divisions may also be subdivided into east-west regions; for instance, the tropical fauna, though homogenous in many characteristics—for example, in the formation of coral reefs—may be recognized as consisting of four parts, namely Indo-West Pacific, Pacific Tropical American, Atlantic Tropical American, and the Tropical West African.
Other faunal areas of the sea are given in fig. 220. These areas are again subdivided as the classification is made narrower to meet more local conditions. The Atlantic Boreal is, for example, divided into east and west sections and the Arctic into High and Low Arctic. For additional details and reference to original data, the student is referred to Ekman (1935). Many animals of eurythermic nature may be quite cosmopolitan and the sublittoral fauna may extend beyond the boundaries shown by the eulittoral animals. See also p. 845 for comparison of thermic boundaries to those of certain faunal regions.
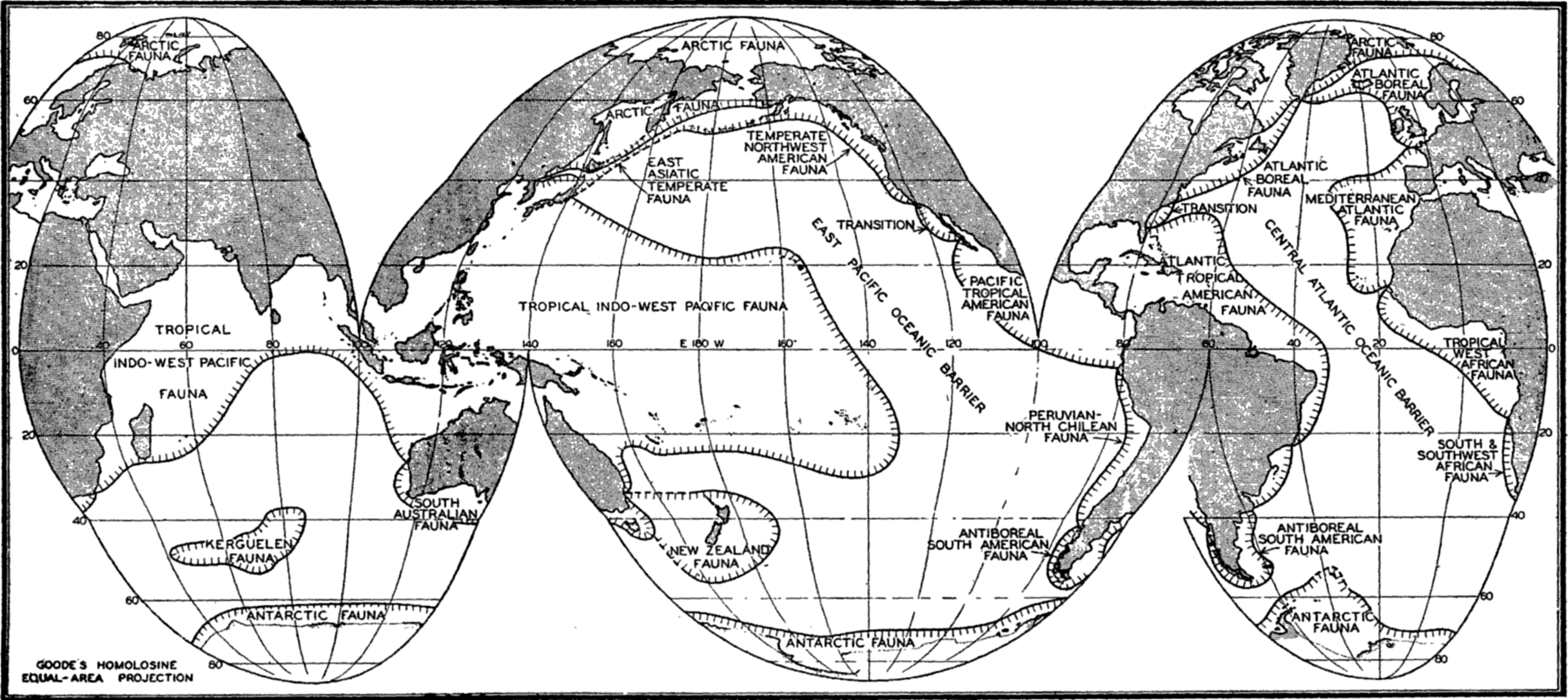
Faunal areas of littoral animals (based on Ekman).
Deep-sea Benthos. In 1843 Edward Forbes, pioneer in marine biology, observed the diminution of the number of animals with increasing depth of water beyond the littoral zone and he established with some hesitation what he called an “azoic zone” covering the deep ocean floor from depths below 300 to 700 m. Before this time, however, in 1819, Sir John Ross reported having found worms in mud brought up from a depth of 1800 m in Baffin Bay, but the idea of life existing in great depths was then so untenable that these and other findings were discredited. It was not until 1860 that positive proof of the existence of animal life in the deep sea was first provided by a broken submarine cable that was brought up for repair from a depth of over 2000 m in the Mediterranean with various bivalve molluscs, gastropods, hydroids, alcyonarians, and worms attached. The dredging operations of the Challenger and other expeditions have definitely shown that benthic animals do live in very great depths, probably in smaller numbers even at the greatest depths, since pressure and cold seem not to be excluding factors. During the Challenger Expedition more than 1500 animal species were discovered below 1000 m and a dredge haul at 6250 m yielded 20 specimens belonging to 10 species. Bottom deposits brought up in sounding tubes from the great deeps contained remains of foraminifera and sponges that probably live at these depths.
The summary in table 94, compiled from Murray (1895) and based on the Challenger observations, will serve to illustrate the vertical distribution of bottom fauna of size sufficiently large to be taken by the apparatus used. The specimens were collected with both dredge and bottom trawl and may therefore include a few not strictly benthic.
All of the animal world below the littoral zone may be spoken of collectively as the deep-sea fauna. Although not many deep-sea collections have been made, the endemic fauna appears to be divisible vertically into two parts, an upper archibenthic fauna (continental deep-sea fauna) and a lower-abyssal fauna, the dividing line between these two being placed at about 1000 m depth.
There is no well-defined border line, of course, between littoral and deep-sea fauna; the border is even less clearly defined between the archibenthic and the abyssal. The natural boundary between faunas is the region of most distinct faunal change. This boundary, however, is influenced by outside factors such as temperature and light, with their
Zone | Number of stations | Average yield of species at each station | Average yield of individuals at each station |
---|---|---|---|
180 m | 70 | 62.8 | |
180 to 900 m | 40 | 51.2 | 150 |
900 to 1800 m | 23 | 30.9 | 87 |
1800 to 2700 m | 25 | 24.0 | 80 |
2700 to 3600 m | 32 | 15.6 | 39 |
3600 to 4500 m | 32 | 10.6 | 25.6 |
4500 m | 25 | 9.4 | 24 |
Outstanding among the eurybathic forms given by Ekman are:
Pennatularia | Kophobelemnon setelliferum | 36 to 3600 m |
Polychaetes | Amphictera gunneri | littoral to 5000 m |
Cirripeds | Verruca stroemia | littoral to 3000 m |
Cumacea | Diastylis laevis | 9 to 3980 m |
Bivalves | Scrobicularia longicallus | 36 to 4400 m |
Snails | Neptunea islandica | 30 to 3000 m |
Starfish | Henricia sanguinolenta | 0 to 2450 m |
Brittle stars | Ophiocten sericeum | 5 to 4500 m |
Sea urchins | Echinocardium australe | 0 to 4900 m |
Sea cucumber | Mesothuria intestinalis | 20 to 2000 m |
That much of the animal life in the deep sea is truly endemic, not merely a downward extension of eurybathic forms, is shown by the presence of vast numbers of species and many genera and higher taxonomic orders that are found consistently only in these deeper zones. Important among the characteristically deep-sea forms are the glass sponges, Hexactinellida, with 15 families, 80 genera, and about 400 species; seven families of Pennatularia; the deep-sea Holothuroidea, of the order Elasipoda with four families, over 20 genera, and many species.
Tables 95 and 96, based on summaries of Ekman, depict further the arrangement of certain elements of the faunas of the three major bathymetric zones.
A striking characteristic of the deep-sea fauna is the relatively smaller number of species in proportion to the number of genera. On the basis of the Challenger data Murray (1913) concluded that the ratio of species to genera decreases regularly from coastal to offshore deep water, so that in the deepest zone the ratio of species to genera is 5 to 4, whereas in the shallow coastal water it is 3 to 1. It is a significant fact that whole orders and numerous families of various taxonomic groups are confined to the deep sea or are characteristic of its population.
Animals | Zones | Number of species |
---|---|---|
Littoral, or littoral and archibenthic | 31 | |
Crinoidea | Littoral to abyssal | 10 |
(North Atlantic) | Archibenthic | 11 |
Archibenthic and abyssal, or purely abyssal | 35 | |
Littoral or mostly so | 13 | |
Starfish | Littoral to abyssal | 28 |
(North Atlantic) | Archibenthic or abyssal | 97 |
Animals | Zones | Number of genera |
---|---|---|
Purely littoral | 62 | |
Littoral and archibenthic | 14 | |
Tunicata | Littoral to abyssal | 11 |
Purely archibenthic. | 3 | |
Archibenthic and abyssal | 4 | |
Purely abyssal | 13 |
Deep-sea animals of the benthic region are in the main mud-dwelling forms adapted in various ways to this mode of life. A considerable number, typified by the isopod genus Munnopsis (fig. 221) and the shrimp Nematocarcinus, are adapted by elongated appendages to the quiet water and the softest of muds; sponges and hydroids are provided with
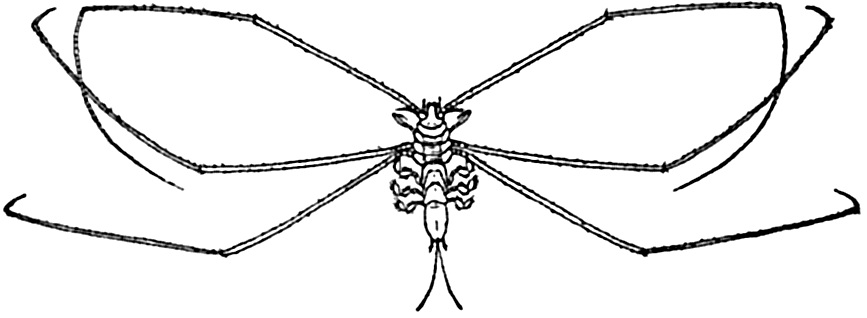
Munnopsis typica, a deep-water isopod (redrawn from Sars).
As previously stated, the population of the benthic region of the deep sea is relatively sparse, the animals decreasing numerically with increasing depth, also with distance from shore. The one factor most operative in limiting the abundance of animal life in the deep sea is undoubtedly food. Abyssal animals do indeed live a precarious life with regard to food. It is not surprising that only relatively few animals have been found on bottoms covered with red clay, for most deep-sea animals depend upon the nourishment obtainable directly or indirectly from the bottom oozes and tests show that the red clay is of all bottom deposits the poorest in organic material. Yet even it is not without its quota of marine metazoan life. It has been pointed out that with the intensified adversity of living conditions in abyssal regions, the animals adapted to survive the physical conditions there would be numerous were it not for a shortage of food. Since plants can live only in the lighted upper strata of the sea, it follows that deep-sea animals are either carnivores or detritus feeders, a population utterly dependent upon plant and animal production in the upper water layers and upon the ultimate sinking of dead bodies of these plants and animals to greater depths. Much of the surface production is broken down, however, by bacterial or autolytic action within the upper or intermediate layers and is thus lost to the dependent abyssal life. The direct food of abyssal forms
It is instructive to note that the poverty of animals in deep-sea zones is apparently not a direct result of depth of water but is intimately connected with distance from continental shores. Murray reports that collections made in depths between 1800 and 3650 m near shore yielded per haul an average of 121 specimens belonging to 39 species, whereas collections made in comparable depths further than about 500 km from shore yielded an average of only 21 specimens and 10 species per haul. This strongly indicates a relative shortage of food for benthic animals in the offshore localities. In the preceding chapter it was emphasized that conditions for phytoplankton production are enhanced in coastal waters where the supply of mineral nutrients in the lighted zone can be enriched through vertical circulation extending to a depth sufficient to tap the store of nutrients that accumulate there through the sinking of organisms produced in surface layers. Owing to the movements of such nutrient-enriched surface water, much of its plankton load becomes deposited in the deep inshore waters and thus supplies more food to the deep-sea benthic animals living there than is possible far from shore. Any offshore hydrographic condition that leads to enrichment of the plant nutrients in the surface layers will produce similar results, reflected in the abundance of benthic fauna that can be supported under such waters. This is illustrated by the surprisingly rich deep-sea benthos encountered by the Challenger Expedition in the deep offshore waters of the Antarctic, especially in the Kerguelen region. Murray believed that this exceptional abundance of deep-sea life resulted from offshore extension of coastal conditions owing to floating ice or to greater destruction of plankton life at the junction of waters of separate origin, but study of the now better-known hydrographic features of the Antarctic reveals that these rich accumulations lie under the region of the great Antarctic Convergence (fig. 158, p. 606) and, according to the investigations carried out by the Discovery, the surface waters of this region show possibilities of a rich supply of nutrients and of a great production of phyto- and zooplankton.
From this dependent relationship it becomes obvious that the deep-sea and abyssal fauna could have come into being only after the pelagic life of the sea had become established, or simultaneously with it. Both the pelagic animals and the deep-sea forms are believed to have been derived from the littoral fauna. The many structural modifications and the numerous endemic genera give evidence that the fauna of great depths is, indeed, an ancient one. But in generalizing, we may say that although the deep-sea fauna possesses many bizarre and unusual forms, nevertheless the structural adaptations found in these animals are only
Horizontal Distribution of Deep-sea Benthic Animals. As a rule deep-sea animals in general are widely distributed, but not to such an extent as was formerly believed would be the case owing to the uniform conditions of the deep-sea environment. The abyssal fauna, however, is the most widely distributed of benthic life, the archibenthic being next, and the littoral fauna the least widely distributed. In other words, the horizontal distribution of marine benthic animals increases directly with increasing depth. Benthic deep-sea genera are usually cosmopolitan, although the species may belong within the limits of certain oceans. Geographical submarine barriers are also influential in limiting distribution. A classical example is the Wyville Thomson Ridge, which forms a barrier between the deep-sea faunas of the Atlantic and the Norwegian Sea, only 12 per cent of the faunas being common to both seas. The reason for this will be discussed further on p. 849.
Nekton, the Swimming Animals
The assemblage of animals comprising this group are provided with efficient locomotive organs enabling them to swim against currents and waves. The locomotor efforts are not only capable of being sustained for considerable length of time, but the movement is also effectively directed towards pursuit of prey, escape from enemies, and instinctive migratory journeys. Structurally, most nektonic animals are well adapted to these ends. They are typically streamlined in shape and frequently covered with slime to decrease resistance in passing through the water. The musculature, nervous system, and sense of vision are notably well developed. Among the members are adult fishes, squids, whales, dolphins, seals, and a few crustaceans. All parts of the pelagic region of the sea contain representatives of the group which, together with the plankton, make up the pelagic life of the sea. It has been noted by Hjort (1912) that the idea of a pelagic mode of life was originally associated with animal life of the ocean surface, but it applies also to the drifting and swimming life of deeper waters, since its main characteristic is its independence of the bottom. Deep-living pelagic animals are called bathypelagic. Pelagic animals are either neritic or oceanic, depending upon whether they belong to the neritic or the oceanic province. There is no well-defined line between the two.
In the nekton we find the great migratory animals which make journeys of hundreds of miles to and from their breeding grounds or roam
The spawning migrations of the European eel, Anguilla vulgaris, are perhaps of the most remarkable among fishes. The journeys involve the swimming of the adult eel over a distance of about 5000 km from European coasts to the spawning area near Bermuda and the subsequent return of the larvae to the fresh-water habitats on the coast of Europe. This will be more fully discussed in a later chapter.
The guiding instinct in these journeys appears more remarkable than that in migratory birds, for recognizable landmarks do not exist in the open sea, where the horizontal chemical-physical gradients are too weak and variable to render anything but negligible directing aids during the oceanic portion of the journey.
Among the marine mammals whales are known to travel great distances. Recovery of American harpoons embedded in blue whales killed in Barents Sea, where harpoons of such make were never used, indicate migrations from the coast of North America (Hjort, 1912). These and other whales alternately travel from low-latitude breeding grounds to the rich feeding grounds of higher latitudes.
The only invertebrates that are clearly nektonic are some of the cephalopods, especially the squids. These animals are powerful swimmers which effect rapid locomotion by spasmodically ejecting jets of water from the mantle cavity through the swimming organ known as the funnel, a tubelike structure representing a modification of the molluscan foot. Associated with this rapid locomotion the cephalopods possess highly developed eyes, and also a highly developed brain as compared with other invertebrates.
A few of the pelagic prawns may be included as nekton, although they are usually on the borderline, approaching either benthic or planktonic life. Such special groups as seals, otters, and marine snakes may for convenience be placed also in this category, although the life habits of some require a period on the immediate foreshore for the care of the young.
Biologically the nekton includes only a very few of the major animal groups, as indicated above; but the large size of individual members, their tendency to form into schools, and their commercial value as food,
Zooplankton, the Floating Animals
The benthic and nektonic groups were the first to be observed or studied by man. But as systematic investigations of the sea progressed, with the improvement of collecting methods and the use of the microscope, it became obvious that another ecological group existed, which for convenience of study should be considered distinct from the bottom-dwelling and the fast-swimming forms to which it holds such vital relationships. This group is now called the plankton, a term first used by Victor Hensen in 1887 to distinguish the vast assemblage of feebly swimming or floating organisms, both plants and animals, that drift about with little or no resistance to water movements. To the plankton belong not only by far the greatest number of marine organisms, but also those of widest dispersal.
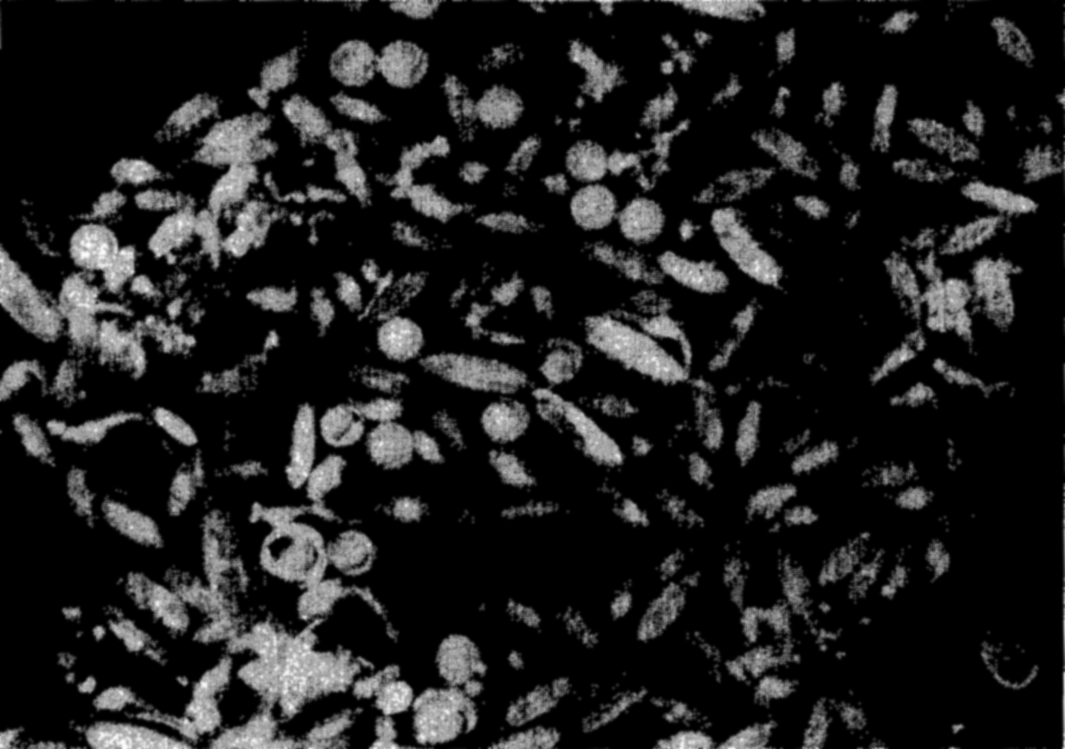
Photomicrograph of a plankton community containing copepods (Calanus and Metridia), young euphausiids, and fish eggs.
The general plankton is divided into two large groups: Phytoplankton and Zooplankton. To the phytoplankton belong most of the diatoms, dinoflagellates, and other unicellular plants or animal-like plants that are capable of synthesizing food. These, in contrast to the consumers making up the zooplankton, are the chief producers of the primary food of the sea. They are more fully discussed in chapters XVI and XIX.
In the zooplankton are included many of the protozoa, especially tintinnids, radiolarians, and foraminifera, a large number of the small crustaceans such as copepods, ostracods, euphausiids, amphiopods; the jellyfishes and siphonophores; many worms; a number of molluscs, such as the pteropods and heteropods; and the eggs and larval stages of most of the benthic and nektonic animals of all kinds. Figures 222 and 223 are photomicrographs of typical zooplankton catches from coastal waters.
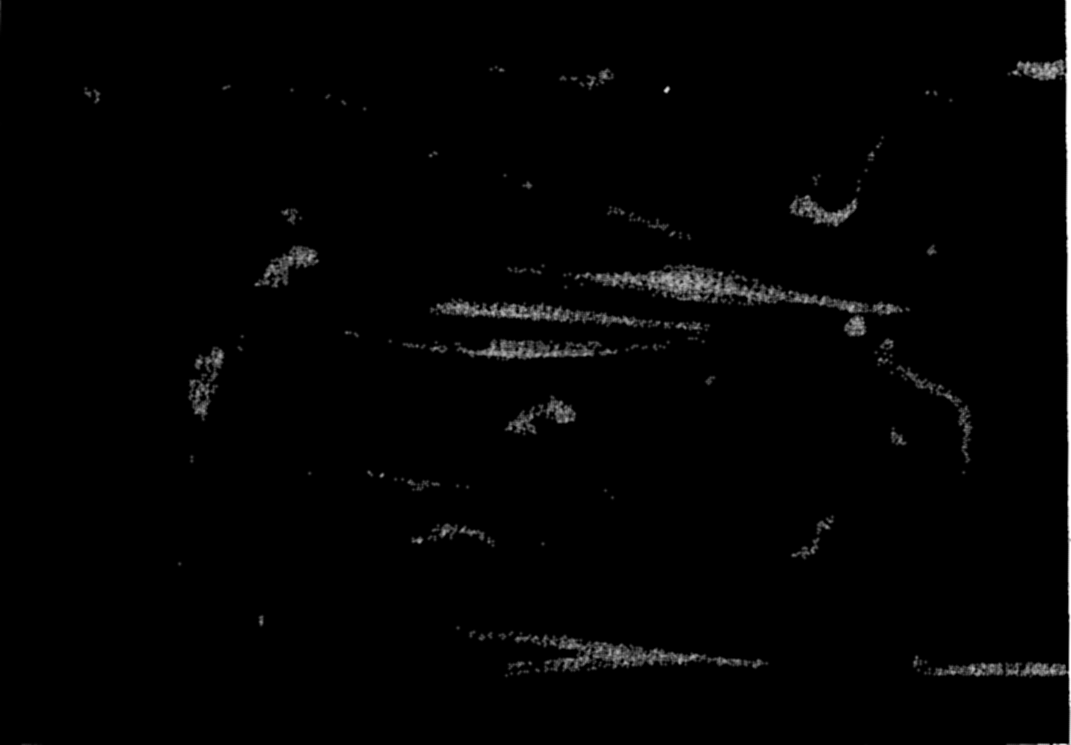
Photomicrograph of plankton dominated by the arrow worm, Sagitta, and including copepods, young euphausiids, and fish larvae.
Undoubtedly the first notice taken of the general microscopic plankton was not of the individual organisms themselves, but rather of such phenomena as discoloration of the water, associated with their swarming. These observations, of course, could only increase the mysteries of the sea, for the causes were in most instances entirely unknown. Probably one of the earliest references applying to general plankton phenomena was made by Pytheas in the fourth century B.C. During a voyage in the North Atlantic he reported that the sea became sluggish and thick like a jellyfish (Herdman, 1923). There are records where the occurrence of the color phenomenon was accompanied by considerable destruction of marine life along the coasts, such as has been witnessed on the coasts of California, Japan, and elsewhere. The terms “red-water,” “red-tide,” and “sliming” have been applied to some of these displays. In other instances, the discoloring became associated with good or bad fishing conditions, and recent investigations (Hardy et al, 1936) have, indeed, shown that there is a correlation between herring catches and color of water resulting from microorganisms (p. 907). Perhaps the most
Among the first important typically planktonic organisms to be studied in detail were the boreal copepod Calanus finmarchicus (fig. 227-c), described by Gunnerus under the name Monaculus finmarchicus in 1765, and Ceratium tripos (fig. 74-a), a dinoflagellate described by O. F. Müller in 1777. Little was accomplished, however, until about 1846, when Johannes Müller introduced the plankton net for use in extensive studies. Since then the plankton has been the subject of a vast amount of investigation.
It will be noted that the three categories, benthos, nekton, and plankton, are not sharply separated. There are not only transitory stages but also many organisms of borderline habits. Most members of the nekton and benthos are for a period properly plankton. The swimming powers of many animals put them midway between the plankton and the nekton, and many forms, for example some mysids, amphipods, cumacids, and so forth, live both on or near the bottom and are sometimes called hypoplankton. Many of the planktonic animals do swim freely and may quickly move many times their body length, although, owing to their small size, little distance is covered. The direction of swimming is frequently haphazard and intermittent, resulting in little progress. However, under certain directing stimuli such as light and gravity, the resultant movements do bring about consistent, although restricted, migrations.
Categories of animal plankton are defined according to duration of the life cycle in the pelagic state, according to size, or according to habitat.
Temporary Plankton. The planktonic eggs and larvae of the benthos and nekton make up what is known collectively as the temporary plankton (fig. 224). This temporary element, or meroplankton as it is sometimes called, is especially abundant in the neritic waters and is composed mainly of developmental stages of the invertebrates, but includes also the young of the fishes.
The following examples give the computed maximum concentrations of various invertebrate larvae taken at separate stations by means of a No. 20 plankton net towed vertically from 25 to 0 m in neritic waters of the Bering Sea during August (Johnson, 1937):
Larvae | Number per cubic meter of water |
Ophiopluteus. | 1,235 |
Echinopluteus | 12,195 |
Bipinnarian | 837 |
Annelid | 8,130 |
Barnacle nauplius | 694 |
Pelecypod veliger | 17,073 |
Gastropod veliger | 7,883 |
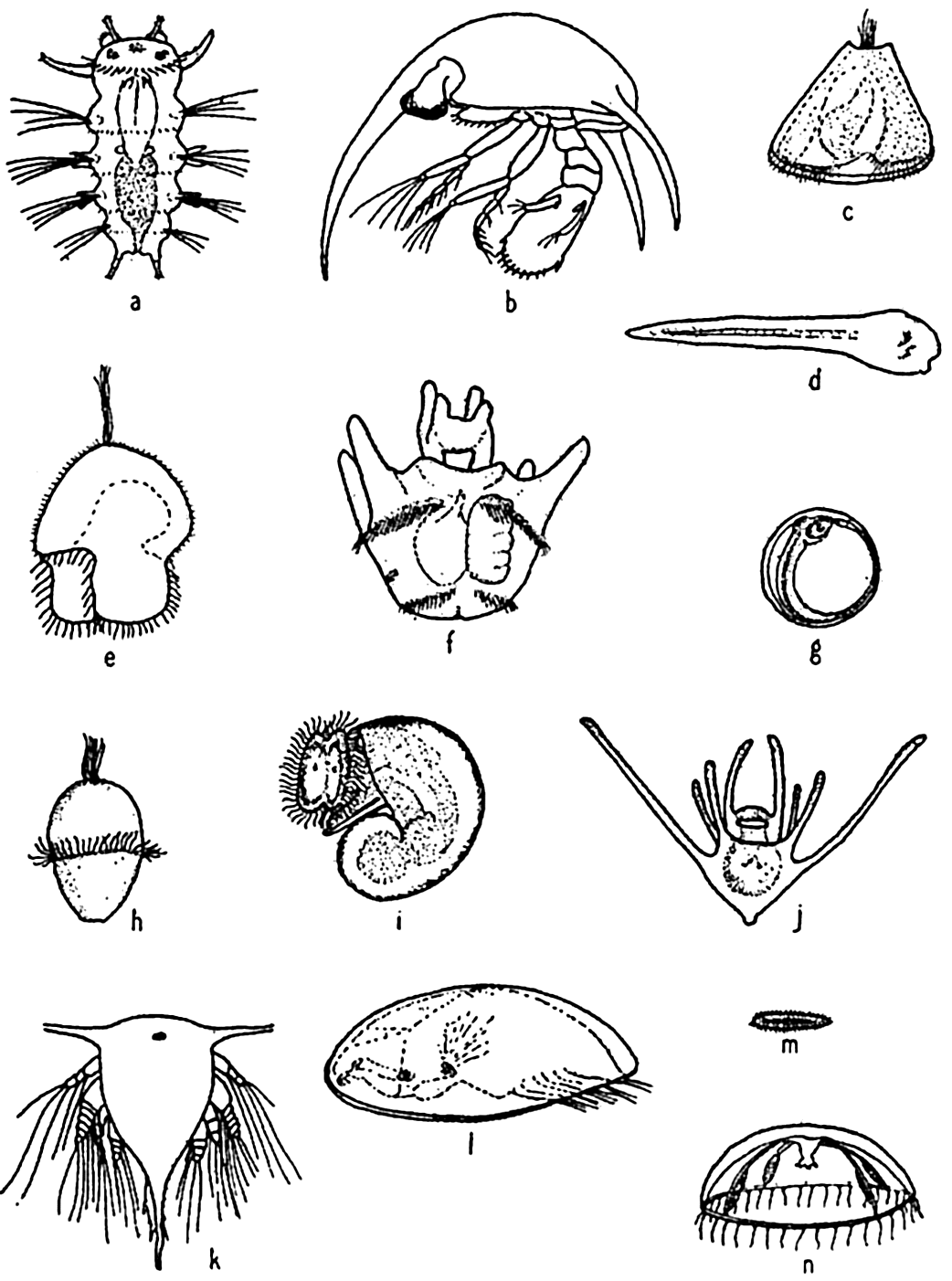
Characteristic larvae of the meroplankton: (a) chaetate larva of the annelid Platynereis agassizi; (b) zoea of sand crab, Emerita analoga; (c) cyphonautes larva of bryozoa; (d) tadpole larva of sessile tunicate; (e) pilidium larva of nemertean worm; (f) advanced pluteus larva of sea urchin; (g) fish egg with embryo; (h) trochophore larva of scaleworm; (i) veliger larva of snail; (j) pluteus larva of brittle star; (k) nauplius larva of barnacle; (l) cypris larva of barnacle; (m) planula larva of coelenterate; (n) medusa of hydroid.
The temporary plankton is characteristically seasonal in occurrence since it is dependent upon the spawning habits of the parental stock. But there is sufficient variation in spawning time of different species, or even continuous spawning of a single species, to provide a greater or smaller amount of temporary plankton at all seasons, even in high boreal waters where the temporary element is usually much suppressed. The length of larval period is also important in this respect, and may vary
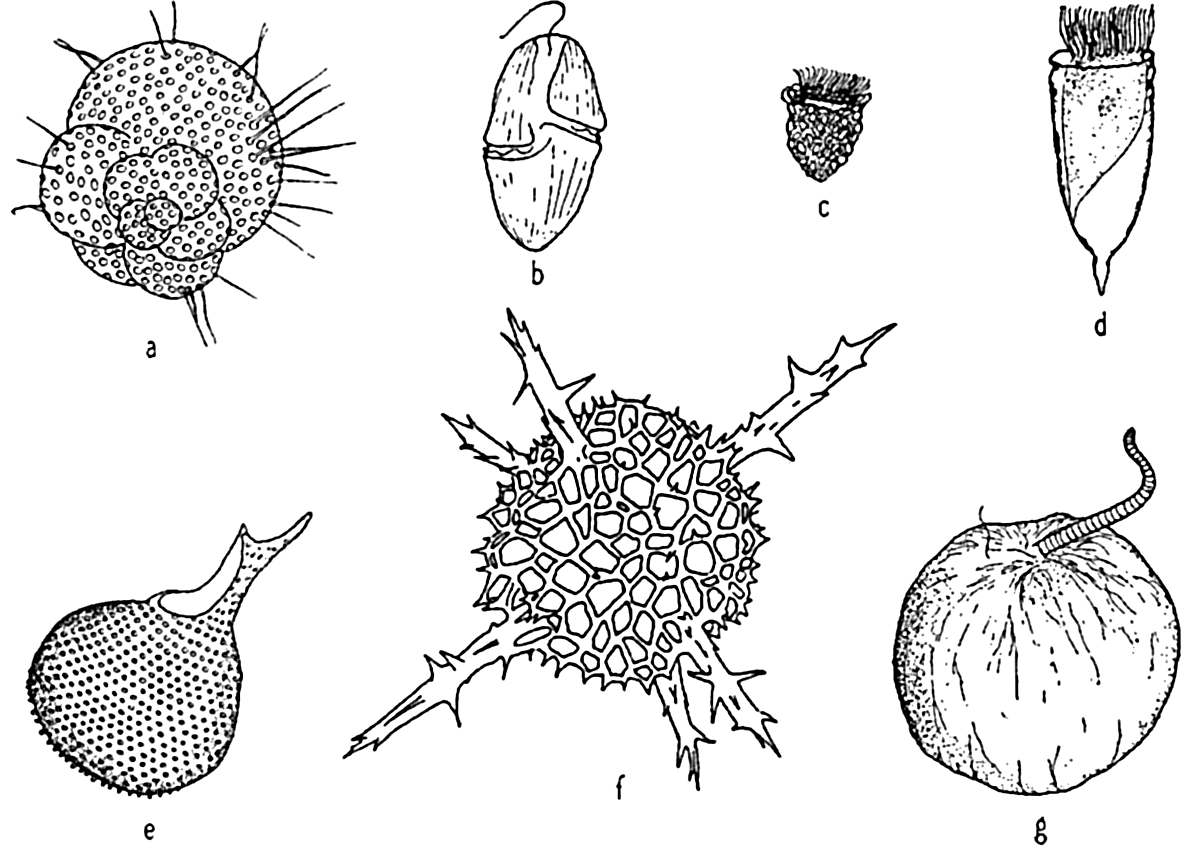
Characteristic holoplankton protozoa: (a) foraminifera (Globigerina); (d) dinoflagellate (Gymnodinium); (c) tintinnid (Stenosomella); (d) tintinnid (Favella); (e) radiolarian (Protocystis); (f) radiolarian; (g) dinoflagellate (Noctiluca).
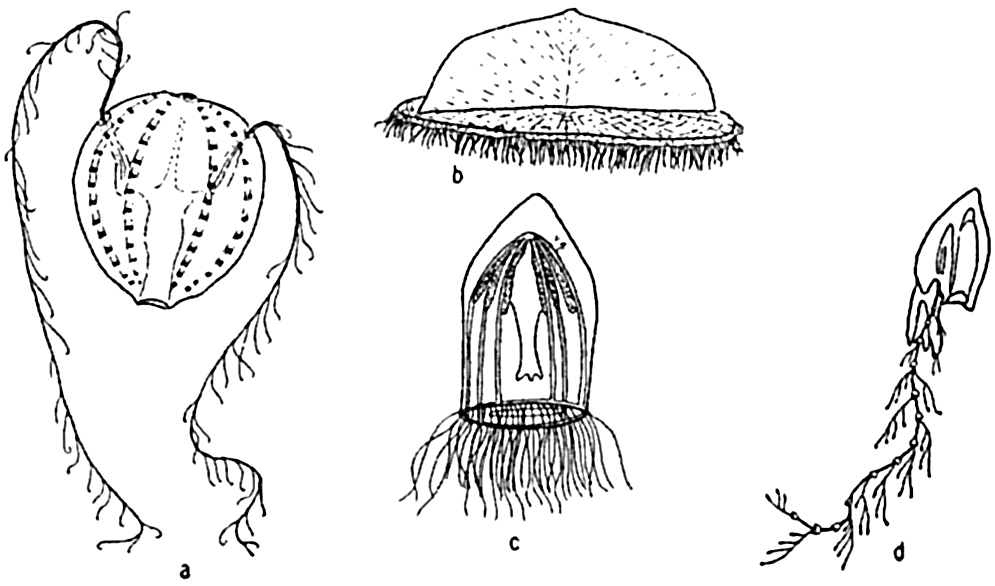
Characteristic holoplankton coelenterates and ctenophores: (a) comb-jelly (Pleurobrachia); (b) siphonophore (Velella); (c) jellyfish (Aglantha); (d) siphonophore (Diphyes).
Permanent Plankton. The remaining part of the plankton is made up of animals living their complete life cycle in the floating state and is called the permanent plankton or holoplankton (figs. 225–228).
The holoplankton is composed of forms representing nearly every phylum of the animal kingdom with the exception of the sponges, bryozoans,
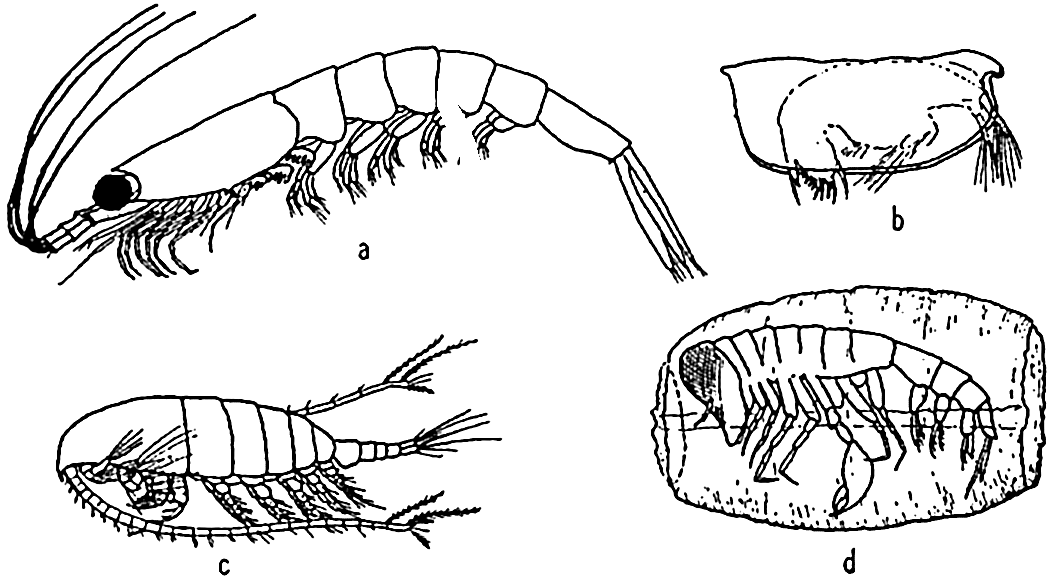
Characteristic holoplankton crustaceans: (a) euphausiid (Euphausia); (b) ostracod (Conchoecia); copepod (Calanus); (d) amphipod (Phronemia) in empty mantle of the pelagic tunicate Salpa.
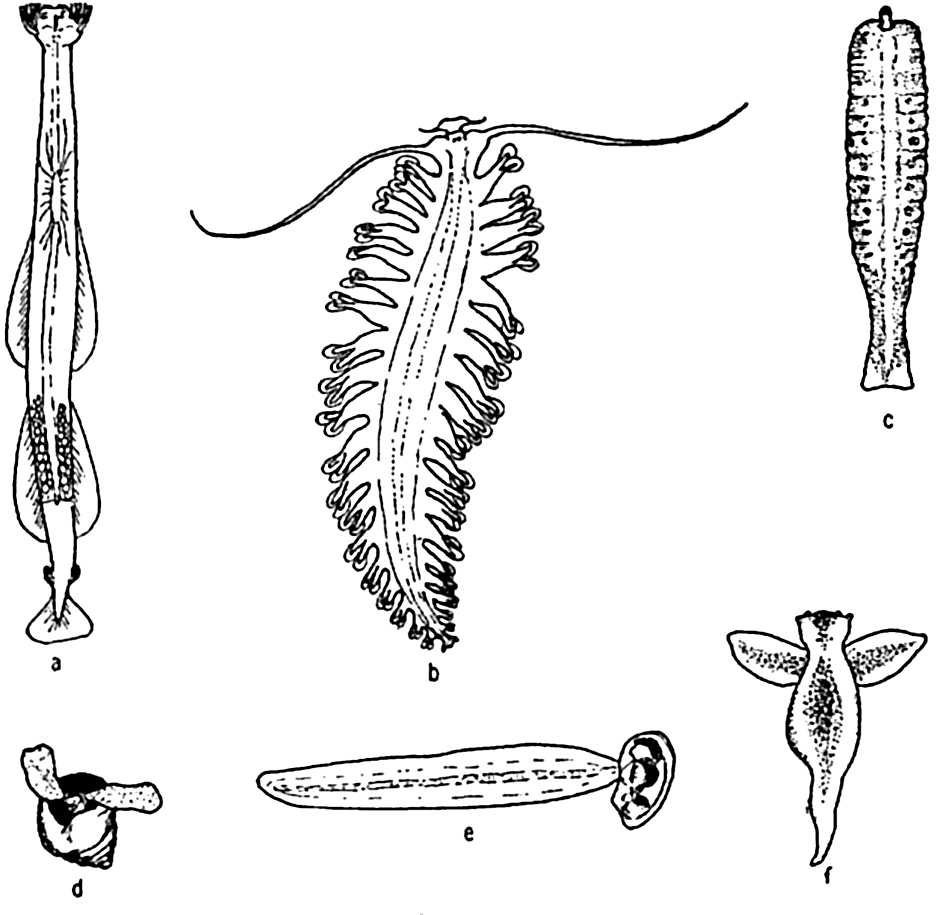
Characteristic holoplankton, miscellaneous: (a) arrow worm (Sagitta); (b) annelid (Tomopteris); (c) nemertean (Nectonemertes); (d) pteropod mollusc (Limacina); (e) tunicate (Oikopleura); (f) pteropod mollusc (Clione).
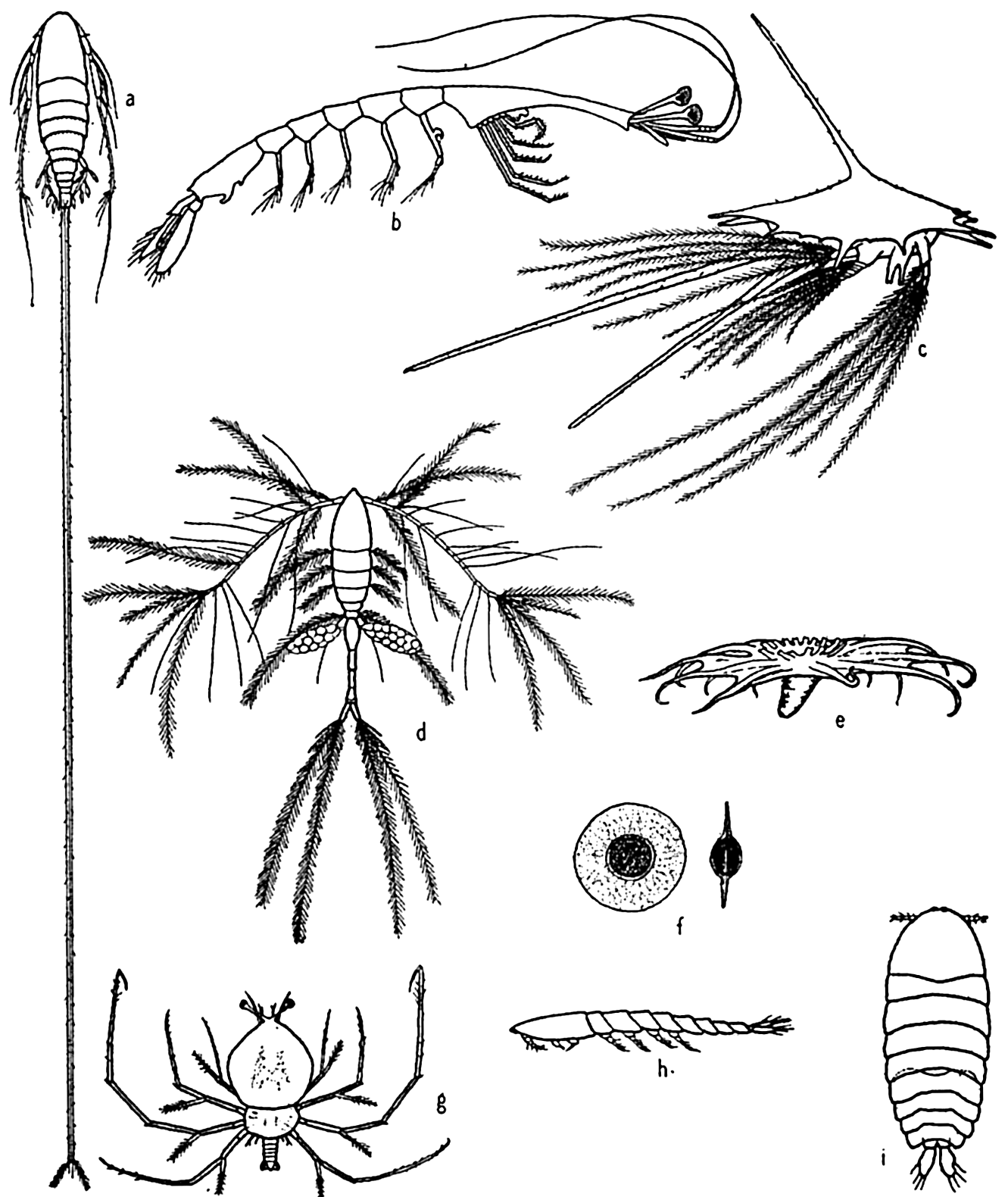
Some floating adaptations of plankton animals: (a) copepod (Aegisthus); (b) decapod (Lucifer); (c) barnacle nauplius; (d) copepod (Oithona); (e) holothurian (Pelagiothuria); (f) pelagic egg of copepod (Tortanus); (g) phyllosome larva of lobster; (h, i) copepod (Sapphirina), side and dorsal views.
All other phyla are abundantly represented in the holoplankton. However, no plankton animals play so vital a role in the economy of the sea as do the Crustacea of the phylum Arthropoda, and among these the copepods rank first in most parts of the ocean although in many instances euphausiids are of equal or greater importance as food for the larger plankton-feeding animals (p. 905). Among the higher crustaceans we should mention the decapod crustacean, Lucifer, which, though of small importance numerically, illustrates a remarkable divergence from others of the group in adjusting itself to a floating life (fig. 229-b). It is of interest to note here that in the sea, as on land, it is the arthropods that have gained the greatest diversity and numbers. In the sea the Crustacea are a counterpart of the Insecta on land.
Macro-, Micro-, and Nannoplankton. Most of the planktonic organisms are microscopic or semimicroscopic in size but there are notable exceptions, for instance, among the scyphozoan jelly fishes, some of which may attain 1 m or more in diameter, with tentacles up to 25 m long. This wide range in size has, for convenience of study, led to yet further subdivision of the plankton on the basis of relative size. Thus we may distinguish broadly between three (or more) convenient size groups. No sharp lines can be drawn between these, but usually the macroplankton is that taken with a coarse net. It includes the large forms and many small ones that can be readily seen with the unaided eye, that is, animals of about 1 mm or more in length that would be normally caught with a net of No. 00 or 000 bolting cloth. (The phytoplankton does not usually form a part of this division.) The forms between about 1 mm and 1 cm are sometimes called mesoplankton, but the term should be avoided in this sense because it is also used to designate the general plankton living in mid-depth waters below the epiplankton. The largest of the plankton forms are sometimes called megaloplankton. The microplankton, also in part called net plankton, is that which is composed of individuals below about 1 mm in size, but yet large enough to be retained by a net of No. 20 bolting cloth with a mesh aperture of about 0.076 mm. The nannoplankton (dwarf plankton) comprises many of the very small forms (about 5 to 60 μ) such as the smaller diatoms, dinoflagellates, coccolithophores, protozoans, and bacteria, which readily pass through the meshes of a new No. 20 net (fig. 90, p. 377) and must therefore be collected by centrifuging the water. Sizes below these are the ultraplankton. Lohmann (1903) demonstrated the presence of nannoplankton by using hard filter paper, and also by a unique method in which he examined the tiny forms caught in the filtering apparatus of the “house” of the appendicularian Oikopleura. The appendicularian “house” is a temporary gelatinous structure that serves as a complete investing case which protects the animal and also acts as a filtering apparatus for catching food (fig. 239). In the “house” there is a set of
Neritic and Oceanic Plankton. Many pelagic organisms, both plants and animals, are bound strictly to coastal waters while others occur normally only in offshore waters. These requirements give rise to two divisions of the plankton, based on their relative dependence on the coast.
To the neritic ecological division belong the forms which normally inhabit the waters in the coastal areas and extend only a short distance seaward, depending upon the depth and type of circulation. The neritic forms on the whole prefer relatively warm water during the growing season, with some reduction of salinity. They are usually seasonal in occurrence, especially in the northern latitudes. Vast swarms of pelagic larvae of benthic invertebrates and many fish eggs and fish larvae are characteristic of the neritic plankton. Resting stages occur in the life histories of many of the species, such as the cladocerans Podon and Evadne, and the rotifers. It must not be understood, however, that the neritic plankton is composed chiefly of temporary plankton. On the contrary, the dominant element is often the holoplankton, adults and young, which are bound to the coastal regions for some unknown reason—perhaps by nutritive, physical, or chemical bonds. The copepods loom large among these permanent plankton forms.
In discussing the plankton of the Gulf of Maine, Bigelow (1926) cites certain of the medusae as conspicuous inhabitants of neritic nature. These are the moon jelly Aurelia; the large red jellyfish Cyanea; and the small hydromedusæ Melicertum companula and Sarsia, all of which are budded from sessile stages in the shallow coastal zone. Many other medusae and also pelagic larvae of such animals as shore crabs and molluscs could be added to this list of strictly neritic forms. It is significant to note, as Bigelow has pointed out for Melicertum companula, that some of these pelagic stages are confined mainly to coastal zones because they have originated there from parental stock dependent upon the immediate coast and not because of any inability to withstand oceanic conditions. Some neritic animals may be found in oceanic waters into which they have been carried, but they are relatively or totally sterile, being unable to complete successfully all stages of the life cycle (p. 859).
The neritic forms are the greatest producers of the sea, for in the coastal waters the food materials are most readily available for plants and through these, successively, for small and large animals. Practical evidence of this relation is seen in the fact that most commercial fish are taken in coastal waters.
The oceanic division, as the name implies, includes forms that are contrasted with the coastal population, since they occur typically at some distance from the coast and over profound depths in the open sea. They constitute the so-called “blue-water” or high-oceanic population. The animals of the oceanic plankton are characteristically holoplankton types that are not dependent on the sea bottom or on the proximity of land; indeed, as a rule, they do not survive long if blown by winds or swept by currents into really neritic situations. Characteristic animals of the oceanic plankton are: Velella, which floats in vast swarms at the surface with its oblique sail projecting to catch the surface breeze; the salps; the violet snail, Janthina exigua, which in time of storm drifts to the beaches in company with Velella; many copepods of different genera, Sapphirina, Rhincalanus, Eucalanus, and others. It is not possible to distinguish sharply between the neritic and the oceanic populations, for there is much overlapping at the border line; there are also forms of relatively great ecological valence, that is, forms tolerant of a wide range of ecological conditions, and such forms are to be found indifferently in both coastal or offshore situations. These are the panthalassic types.
Special Adaptations of Animals to Planktonic Existence. In discussing the adaptations of the phytoplankton, it was pointed out that the rate of sinking of a body heavier than water depends upon the ratio of surplus weight to friction, and friction in turn is determined mainly by the surface area. The simplest way to obtain a relatively large area is to reduce the absolute size. Therefore the small size of most planktonic animals is of extreme importance in keeping them afloat; but, as indicated for the plants, special structural adaptations are also provided.
In contrast to most members of the phytoplankton, the planktonic animals usually have some power to swim. That this is highly important, especially for the larger crustacean forms, is shown in the following summary from Gardiner (1933), which gives the average time required for anesthetized Calanus finmarchicus of different lengths to sink through a column of water 250 mm deep having a temperature of 18.5°C and a salinity of 35.01 ‰:
Length of specimen (mm) | Mean time for sinking (seconds) | Length of specimen (mm) | Mean time for sinking (seconds) |
2.1 | 181.3 | 3.2 | 70.3 |
2.3 | 110.6 | 3.4 | 58.9 |
2.6 | 114.9 | 3.6 | 57.9 |
2.8 | 106.8 | 3.9 | 43.9 |
3.0 | 74.3 | 4.0 | 31.0 |
The experimental data included additional lengths, and the correlation between sinking time and length of specimen, expressed as coefficient of correlation, was
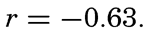
The ability to swim varies greatly with different animals, but even very feeble swimming, when favorably directed as a response to gravity or to light, must work to great advantage in keeping the organism within the water stratum having tolerable living conditions. The ciliates depend largely on ciliary action as do also the ctenophores, the trochophores, and other similar larvae. It is significant to note that the pelagic young of bottom-dwelling forms almost invariabley possess a great development of cilia or a greater length of spines and bristles in greater profusion than occurs in the adults. Euphausiids and at least the larger copepods keep their locomotive organs (pleopods, maxillae, second antennae, and so forth) almost incessantly in motion for feeding, respiration, and swimming, and appear to depend rather little on special suspensory organs. The chaetognaths, or arrow worms, all species but one of which are planktonic, depend mainly upon swimming, for which they are rather well adapted by development of fins and longitudinal muscles. But many of the plankton animals that can swim are also especially adapted to resist sinking when they are in a state of rest. Here the means of adaptation are mostly along the lines of increased length of appendages, of spines or bristles, or of dorsoventral flattening of the body (fig. 229, p. 818).
The radiolaria and pelagic foraminifera develop long spines and irregular shapes, a method not unlike that employed by some diatoms, and the size of some species of radiolaria may be much decreased in the warmer surface water. In Challengeria, for example, certain of the smaller species averaging about 0.11 to 0.16 mm live in the less viscous surface waters of 50 to 400 m depth, whereas the larger species averaging from 0.35 to 0.58 mm live in the colder viscous waters of 1500 to 5000 m depth. Between these depths the average size of different species ranges from 0.26 to 0.28 mm. The chief method of flotation for many of the copepods is found in the increased length of appendages and the spines or bristles that grow upon them. The tropical forms show a greater development of plumose structures than do the northern species, and this is just what we should expect because of the reduced viscosity of the warmer water (see p. 857). The warm-water copepod, Sapphirina (fig. 229h and i), has no extensive development of spines of plumose structure, but it is flattened dorsoventrally in a manner that enlarges the ventral surface and gives greater water resistance while sinking. The lobster phyllosoma larva is similarly constructed and adapted to its pelagic period. The long spines, antennae, and so forth, common in
The presence of oil is a common and most effective method of reducing specific weight in pelagic eggs; it is also found in the copepods, euphausiids, radiolarians, Noctiluca, and others. In the copepod Calanus finmarchicus there is a well-defined hydrostatic organ filled with oil; this consists of a very thin sinus lying in the mid-dorsal axis of the body. The oil also apparently functions as a food reserve since it varies greatly in quantity, and its quantity is perhaps indicative of past living conditions.
Among the siphonophores, pneumatic floats serve as a hydrostatic apparatus. Physalia and Velella are good examples of this type of adjustment and, in addition to the floats, possess sails that extend above the water surface to enlist the aid of the wind in giving more rapid transportation.
Finally, it should be noted that the water content of many animals of the plankton community is very high, being in excess of 95 per cent in some jellyfish. The jelly-like transparency of many plankton animals, for instance the salps, the leptocephalus eel larvae, the pelagic annelid Tomopteris, the ctenophores, the heteropods, the chaetognaths, the copepods Eucalanus and Haloptilus, and many others attest to the high water content of their bodies. The maintenance of this high water content is facilitated because the body fluids are isotonic with sea water. The skeletons (when present) of planktonic animals are remarkably thin in contrast to many related benthic forms. Some of the typical means of flotation are illustrated in fig. 229.
For a fuller discussion of the planktonic adaptations, the reader is referred especially to Steuer (1910).
RELATIONS TO PHYSICAL-CHEMICAL PROPERTIES OF THE ENVIRONMENT
A study of the influence of environmental factors on the lives of marine animals encounters numerous difficulties not met with in the study of plants. The fundamental factor of nutrition is more clearly recognized in plants than in animals, for we know rather well the simple elements that are utilized by plants in the synthesis of their carbohydrates and proteins, and several of these elements are now readily measured quantitatively. The means by which the elements are conveyed to the very limited euphotic zone of the sea where plants may grow can also be determined approximately by hydrographic surveys. The quantity of light needed for plants to balance photosynthesis and respiration is measurable. Under favorable conditions the rate of production in unicellular plants, especially diatoms, is extremely rapid, thus offering a good index to the operation of environmental factors. In most animal
The duration of life of the individual animal is on the whole relatively long, and frequently the development is very complex with separate stages, each with habits and needs of its own (p. 318). The various degrees or methods of locomotion are highly important in the adjustment to the conditions of life. All these variations make it necessary in biological studies to consider not only the separate species but also the whole life cycle of the individuals from the egg to the spawning adult. This will become increasingly obvious as we consider in the following pages the influences of the various factors.
In studying conditions of the natural environment it is not possible to isolate completely for discussion the separate factors, since these factors do not in nature operate separately on the population but rather directly or indirectly in groups. We shall discuss the subject, however, under separate headings in order to emphasize outstanding features, at the same time feeling free to introduce correlated features. These headings are (a) light, (b) salinity, (c) temperature, (d) ocean currents, (e) oxygen.
Light
Of all the environmental factors, light is perhaps the most readily isolated of all, for its momentary influence on temperature is small and therefore of small importance in influencing circulation or density of the water. Its direct effect on chemical reactions in the sea is concerned largely with metabolism of the organisms, as in photosynthesis and pigmentation. Light is also a factor showing very frequent and marked fluctuations in the upper layers of the sea.
To animals, light has its greatest significance indirectly, as a source of energy for the photosynthetic processes of plants upon which all animals are wholly dependent for their nourishment. Aside from this, animal life may exist without solar light, as is witnessed by the presence of animals in the abyssal depths. Nevertheless, light or absence of light has been one of the most potent factors in the molding of structural development and in the adaptations of most marine animals. Light is also a great factor in the movement of animals.
Light and Color. The variegated colors, bandings, mottlings, and so forth, of many marine fishes living among the fronds and stipes of sea-weeds is a well-known phenomenon to anyone familiar with the sea. Likewise, various shades and patterns of gray and black are common, for instance, among tide-pool sculpins and the littoral flatfish of sandy or muddy bottoms. Many littoral crustaceans living upon seaweeds
In more recent publications Sumner (1934, 1939, 1940) has reviewed his own and other investigations pertaining to mechanisms of color changes and quantitative effects of visual stimuli on pigmentation, especially in fishes. The mechanisms that bring about these changes in color or shade are associated with the chromatophores or pigment cells, the contents of which are either activated directly by intensity of incident light or mediated through the eyes and nervous system in response to color or to albedo (proportion of incident light reflected) of the background. The pigments of the chromatophores produce degrees of shade or color by the dispersion or concentration of the pigment within the irregularly shaped chromatophore cell. A display of greater or smaller amounts of the pigment results, either evenly over the whole surface of the body or irregularly in patches, the resultant effect depending upon the degree of dispersion or concentration of pigment and upon the amount of pigment involved.
In the above we have referred mainly to a method of color change that is concerned only with rearrangement of the pigments already present. Such changes are usually quite rapid and may even occur within a few seconds, as is witnessed by reactions of the cephalopods in which color intensity may quickly shift from pale gray to a rich chocolate as the animal passes over a lighter or a darker substratum. Here light appears to be the stimulus activating the changes but, in addition, nervous reactions related to feeding or presence of enemies may also produce the temporary color changes in cephalopods.
A second type of color change involves gradual change in the quantity of pigment or in the number of chromatophores present. Experiments by Sumner and Doudoroff (Sumner, 1940) showed that during a period of about two months the amount of the pigment melanin produced in the guppy (Lebistes reticulatus) was nearly independent of the intensity of the light but was dependent upon the albedo of the background. The amount of melanin (or the number of melanophores) was found to be
Much might be written pertaining to protective coloration of animals, its physiology, evolution, and purpose, but ecologically the greatest significance that can be attached to the ability, possessed by some animals, to respond to their background by closely matching their own color with it, is the protection they must thereby gain in relation to their organic environment. It has been shown experimentally (Sumner, 1935) that certain fishes (Gambusia) adapted to blend with their background have a greater likelihood of survival from attack by predators than do fishes of the same type that are in contrast with the background. If we may trust our own visual sense as an index, we know that only the closest scrutiny can reveal the presence of some animals made practically invisible by the cloak of blending color or shade.
In what has been said, we have considered only littoral animals that live on or near a more or less illuminated background of solids, either the substratum or larger plants. There is, however, a great array of animals not normally provided with such a background. In the pelagic habitat, for example, the “background” is the surrounding water, and in the abyssal-benthic division the background is entirely devoid of solar light. In the pelagic division the aqueous surroundings have all degrees of light intensity, from sunlight at the surface to absolute darkness in the great depths. The quality of light is also different at different depths, since water does not absorb all light rays uniformly, but the Michael Sars Expedition found that in southern waters even the most penetrating rays failed to affect photographic plates exposed for two hours at a depth of 1700 m (p. 83).
It is of special biological interest to note that at a depth of 500 m the rays detected are not entirely diffused by the water but are directed so that shadows, however faint, should result. The dorsal side of an animal even at this depth is more exposed to light than the ventral or lateral sides and this may exert an influence, along with the force of gravity, in the orientation of the animals. To animals living at shallow depths the direction of light appears to be of great significance and most fishes of these zones are notably darker above than underneath. Investigators concerned with the effect of light on pigmentation have expressed the opinion that the shade assumed by an animal is chiefly determined by the ratio between the light from overhead and the light reflected by
Just how this principle of reaction to light would operate to affect the fishes and other animals in the open sea has not been shown, but in the zone where there is still considerable light there must be a difference in the light intensity from above and below. It appears that the above principle is best applied to conditions where the water is shallow and the background a well-defined reflecting surface with various values for the albedo. Be this as it may, there is still much evidence, especially from the findings of the Michael Sars Expedition in the North Atlantic, to show that there is a very real, though not satisfactorily explained, correlation between the light entering the sea and the color of the animals at various levels of light penetration.
The uniform dark pigmentation of fishes in the lightless depths, as contrasted with the ventral-dorsal differentiation of those inhabiting the lighted zone, indicates that the direction of light is significant in the distribution of pigment. But just why abyssal fishes do not become blanched in the absence of light as do cave fishes has not been satisfactorily explained.
It is generally known by mariners and fishermen that the animals living at or near the surface of the sea, especially in lower latitudes, are commonly blue in color. Such common fishes as the flying fish, mackerel, bonita, tuna, and others have backs of blue or blue-green. Some invertebrates, for example the siphonophore Velella and the pelagic molluscs Janthina exigua and Glacucus eucharis, are among the larger and better known, while copepod species of Pontellopsis, Anomalocera, and Corycaeus are frequent among the microscopic forms.
The blue and green colors are indeed striking characteristics of the surface open-sea life but it must not be concluded that all oceanic animals are blue or even predominantly so with respect to numbers. In many of the smaller forms, copepods and other crustaceans, pale red is a very common color, which like blue may occur as faintest tints in relatively hyaline forms. Hyaline or colorless animals (sometimes with the digestive tract or other parts colored) are as characteristic of the open sea as are the blue, or even more so, from near the surface to moderate depths (up to 200 to 300 m or deeper). Among these hyaline forms may be mentioned the salps Salpa and Doliolum; the arrow or glass worms Sagitta; the pelagic heteropod molluscs Pterotrachea and Atlanta; the Ctenophora or comb jellies; the copepods Eucalanus and Haloptilus; and many fish
larvae. The list could be extended almost indefinitely. The crystal clear aspect of many of these animals must make them practically indistinguishable to predators. Yet if this is a protective adaptation, we are left with the need of explaining why many species of these hyaline forms are so rare in comparison with others of the same type of animals that are more conspicuous owing to presence of color. As examples we may mention the hyaline versus the opaque or colored copepods. The latter are far more abundant as a rule. Perhaps the protective feature is coupled with a low rate of fecundity; or in plankton forms the unselective mode of feeding by predators may be yet another explanation since the hyalinity would be of little survival value.
At depths of about 300 to 500 m the silvery fishes, Argyropelecus (fig. 231-a), Chauliodus, and others, form a conspicuous though small part of the fauna, and there is a marked increase in reddish and dark-colored animals: dark fishes such as Cyclothone; many red crustaceans, wholly or partly colored, including, for example, the red prawn Acanthephyra, and numerous copepods such as Getanus, Metridia, Pleuromamma, and Euchaeta. The red Sagitta macrocephala and Eukronia fowleri of these depths are in contrast with colorless chaetognaths taken at higher levels. Other dark colors occur, including dark violet pteropods (Peraciles diversa) black or dark violet fishes (Gonostoma spp.), and brown medusae (Atolla).
In the deeper lightless waters, brilliant red, scarlet, or red-violet prawns (Acanthephyra spp.) persist, but the fishes are all black, black-violet, or brownish.
The depths at which these characteristically colored animals appear are not the same in all parts of the world. In waters of higher latitude the species of intermediate depths are nearer the surface than in tropical seas. Forms occurring at about 200 to 500 m in the higher latitudes, between about 67° and 50°N, will be found near 750 m at the latitude 33°N (Hjort, 1912). This difference indicates strongly a correlation with the degree of submarine illumination, for the upper limit of the dark pigmented forms agrees with the relative depths of light penetration at these latitudes. Further evidence is gleaned from the fact that in related species or varieties the darker and more heavily pigmented ones come from the greater depths. For example, among the fishes the light-colored Cyclothone signata has its lower limit near the upper limit of the black C. microdon. This is indicated by the following hauls taken at three depths by the Michael Sars at about 35°N.
Depth (m) | Light-colored C. signata, individuals | Dark-colored C. microdon, individuals |
---|---|---|
500 | 1240 | 214 (small) |
1000 | 82 | 448 |
1500 | 22 | 322 |
A similar vertical distribution was found for certain other forms of life. The deep-sea medusa Atolla bairdi has four varieties based on the degree of pigmentation. They range from a variety with pigment only in the stomach to the deepest dwelling variety with quite general pigmentation.
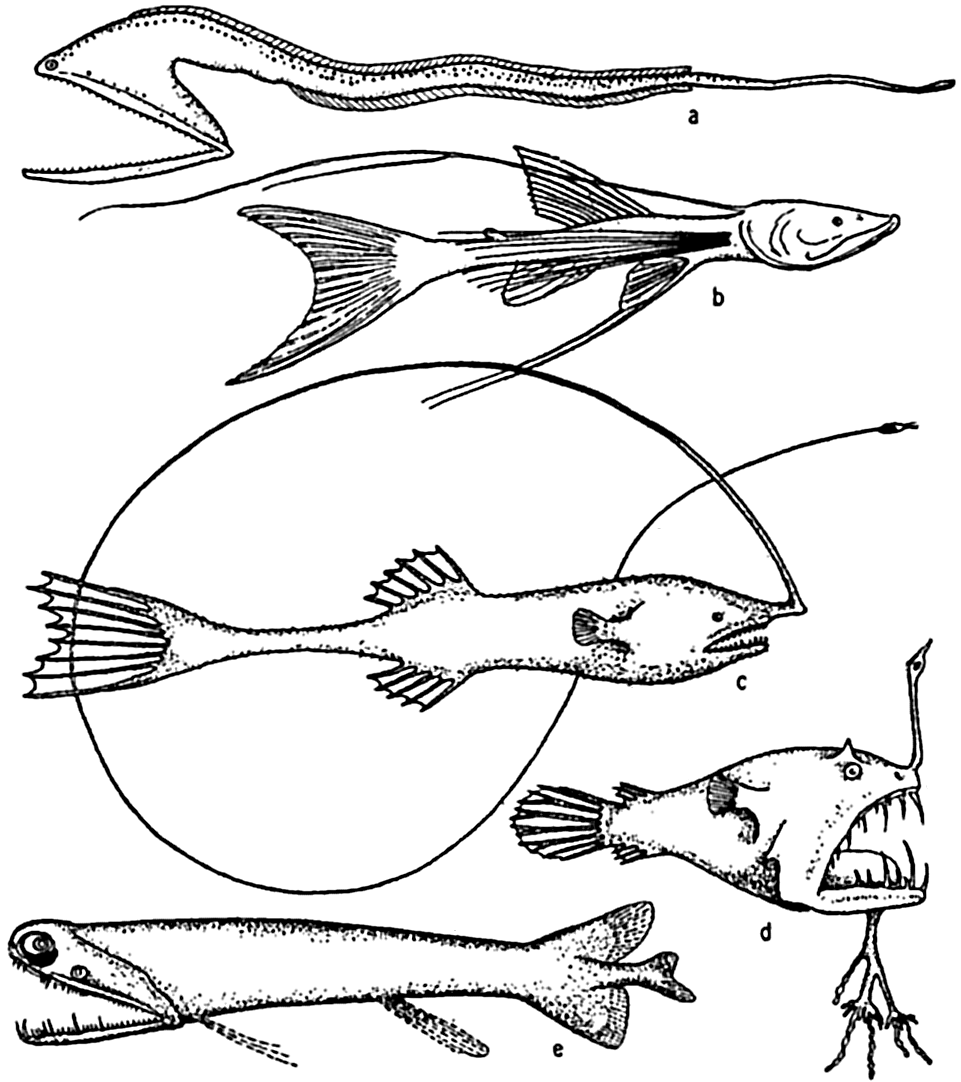
Deep-sea fishes: (a) Macropharynx longicaudatus (after Brauer), length 15.1 cm, from 3500 m depth; (b) Bathypterois longicauda (after Günther), length 7.6 cm, from 550 m depth; (c) Gigantactis macronema (after Regan), length 13.3 cm, from 2500 m depth; (d) Linophryne macrodon (after Regan), length 5.3 cm, from 1500 m depth; (e) Malacostus indicus (after Brauer), length, 8 cm, from 914–2500 m depth.
The red rays in the spectrum are quickly absorbed by the surface waters, hence, the animals below the surface live in a green, blue-green, or lightless world. In the abyssal regions it is indeed difficult to imagine what survival value any color can have, but near the surface, for example, the fishes with blue or green backs and silvery or light ventral sides are rendered thereby less conspicuous both from above and below, and the darker colors of fishes in dimly lighted depths also seem appropriate. In daylight red is a conspicuous color, but in the sea a red object appears quite black at a distance of only a few meters owing to the rapidity with which red rays are absorbed by water; and at depths below the penetration
Light and Structural Adaptations. That the quantity or quality of light has a marked influence on the coloration of marine animals inhabiting different depths of the sea seems beyond all reasonable doubt, but the influence of deep twilight or absence of light has indirectly placed a more remarkable stamp on structure than on color. This is manifest by the strange and weird anatomical adaptations, especially of many abyssal fishes.
These adaptations are concerned with structural modifications fitting the animals better to survive in faintest light or in utter and perpetual darkness. They are mainly along three lines: (1) tactile structures, (2) food-procuring contrivances, (3) light production.
Reference to publications dealing with abyssal fishes (Günther, 1887, Brauer, 1906, Murray and Hjort, 1912, Regan, 1932) will illustrate how characteristic are modifications along these lines. Development of tactile devices is an important feature in numerous instances and is well illustrated in Macropharynx longicaudatus, several species of Bathypterois, Gigantactis macronema, Linophryne macrodon, and other deep-sea angler fishes (fig. 230). These sensory structures may, as indicated in the figures, involve modification of the whole body whereby it is elongated and ends in a disproportionately long threadlike tail as in Macropharynx; or, in the more conventional bodies, the fin-rays may grow to a length several times that of the body; or tactile threads of various types may grow directly from the head. The prawns of deep water also possess extremely long tactile antennae, some of which may be twelve times the length of the body as in Aristaeus aristaeopsis.
The food-procuring contrivances of deep-sea fishes consist commonly of an extraordinarily immense mouth in proportion to the body size. The stomach and abdominal wall may be so elastic that it is possible for some of these fishes, Cheasmodus or Melanocetus for example, to swallow other fishes three times their own size and many times their own weight. Many of the deep-sea fishes are provided with formidable teeth, attesting to their carnivorous nature. As if this were not enough, some species of deep-sea angler fishes possess a tactile organ, the illicum, useful as rod and line, and provided with a terminal luminous lure, which in some cases may even be armed with hooks, as is the case with Lasiognathus (Regan, 1926; fig. 231-b). These angling processes may be four times as long as the fish, as in Gigantactics macronema (fig. 230-c). In food habit these fish must be mainly piscivorous. The possession of these unusual devices is correlated with the scarcity of food in the deep-sea pelagic
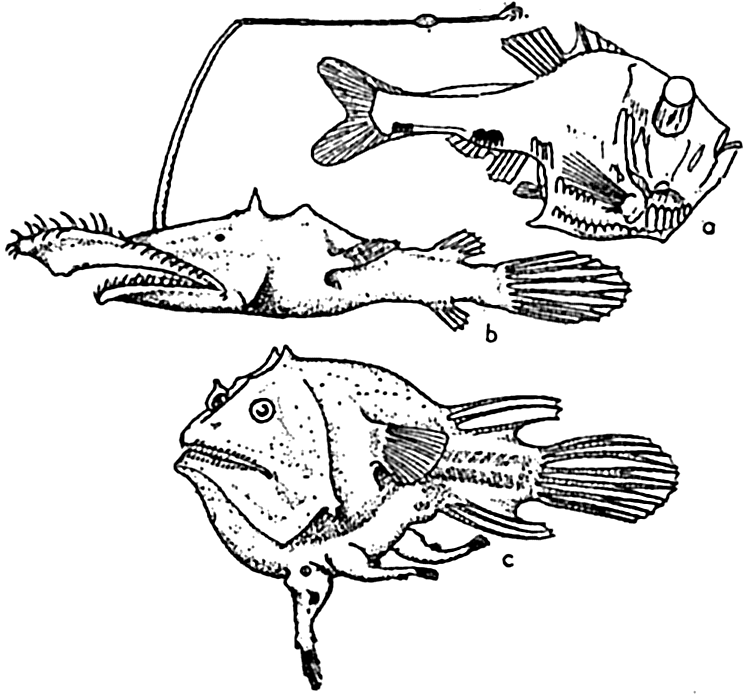
Deep-sea fishes: (a) Argyropelecus hemigymnus (after Hjort), length 3.5 cm, from 300 m depth; (b) Lasiognathus saccostoma (after Regan), length 7.5 cm, from 2000 m depth; (c) Edriolychnus schmidti (after Regan), length 7 cm, from 2000 m depth.
We have but little information regarding the depth at which fishes and other animals can see, but for purposes of comparison the question may be raised as to the depth at which light is still perceptible to the human eye. The human eye appears to respond to an intensity of light about 10−10 times that of bright sunlight, the greatest sensitivity at low intensities lying in the green at wave length .503 μ. One can easily find the depth at which light is still perceptible by making use of the absorption coefficients at .503 μ which have been found for the different types of water (p. 84). These depths, based on Utterback's data (1936), are entered to the left in table 97, and to the right in the same table are entered corresponding values which Clarke has computed from his observations. In the clearest oceanic water light should still be perceptible at a depth of 700 m, whereas in average oceanic water the corresponding depth would be 300 m, and in turbid coastal water 60 m. The values agree well with those of Clarke (1936), who finds that in the Sargasso Sea light is perceptible down to 430 m, whereas in Woods Hole Harbor light could not be perceived below a depth of 75 m if the waters were that deep. Clarke compares his results with Beebe's observations from the bathysphere, according to which complete darkness for the human eye was reached at a depth of between 520 and 580 m. Helland-Hansen's observations indicate that even at these depths light is directed, wherefore weak shadows will be present. According to the experiments by Grundfest (1932), the sunfish Lepomis can see at illumination as low as that
Type of water (from Utterback's data) | Depth (m) | Region (from Clarke) | Depth (m) |
---|---|---|---|
Pure sea water. | 1530 | ||
Clearest | 700 | Sargasso Sea | 430 |
Oceanic water Average | 300 | Deep basin, Gulf of Maine | 230 |
Turbid | 158 | Georges Bank | 180 |
Clearest | 115 | Off Gray Head | 130 |
Coastal water Average | 80 | Woods Hole Harbor | 75 |
Turbid | 57 |
In a world of much reduced light or absolute darkness, it is to be expected that the organs of vision will be among those modified, either towards ends resulting in better utilization of the faint rays of solar or biological light that may be present, or in diminution or entire loss through disuse. Both of these modifications have occurred, even in forms that are closely related. The former has been accomplished in some instances by enlargement of the eyes to receive better the faint rays of light, and by increase of visual cones and loss of the rods in the retina. The cones are the functional cells for dim light. Reduced light appears also to have led in some instances, in both fish and cuttlefishes, to the development of “telescopic” eyes, that is, large-lensed eyes that protrude from the sockets as in Argyropelecus (fig. 231-a), indicating binocular vision. This type of adaptation is not an isolated one but is said to occur in five orders and several suborders of fishes.
The conditions of light in the deep sea have not invariably led to a convergent evolution providing more efficient eyes according to the above modifications; many animals, especially the bathypelagic fishes (but also crustaceans, at least one species, and a squid), have evolved species that are blind or have degenerate eyes, apparently atrophied from disuse.
Bioluminescence. In the biological world, heat and motion are common expressions of energy but electricity and light are also produced, especially by marine animals. Bioluminescence, popularly spoken of as “phosphorescence” because of an earlier belief that it was caused by the element phosphorus, is primarily a phenomenon of the sea. It is not exclusively confined to the sea, to be sure, but is overwhelmingly more abundant there than anywhere else. No light is known to be produced by fresh-water animals with the exception of one aquatic glowworm, and in terrestrial organisms the production of light is relatively rare. In the sea, the power to emit light is not confined to any particular taxonomic group of animals but is so generally distributed that most major animal groups are involved, from the protozoans to the vertebrates. Many marine bacteria also are luminescent.
The number of animal species capable of emitting light runs into many thousands, distributed among such groups as the radiolarians, dinoflagellates, hydroids, jellyfishes, alcyonarians, ctenophores, bryozoans, polychaete worms, brittle stars, many crustaceans (including ostracods, copepods, schizopods, and decapods), gastropods, bivalves, cephalopods, protochordates, and true fishes. Harvey (1920, 1940) should be consulted for a fuller discussion of these. It is highly probable that many groups not now known to be luminescent will prove to be so as our information becomes more complete. Only recently the nemerteans have been reported to have members that luminesce. Light producers are found in all marine communities from the surface of the sea to abyssal depths. As far as surface display is concerned, luminescence is more common in tropical than in northern latitudes, although it may be very striking in boreal waters during the warmer seasons. But this is associated in part with the fact that many luminescent organisms of the surface, especially the dinoflagellates, are mainly thermophiles, requiring
The light rays produced by organisms are wholly within the range of human vision and may at times be sufficiently brilliant to make the crests of breaking waves, the wake of a ship, or other mechanically agitated water glow with a general greenish light of sufficient intensity to enable one to read. This general phenomenon wherein the water itself appears to glow is caused by innumerable microscopic organisms, mainly dinoflagellates of various types, such as Ceratium or Noctiluca (fig. 225-g). Scattered throughout the glowing water are the larger, brighter points of flashing light emitted by jellyfish, copepods, euphausiids, annelids, or other larger forms.
In many of the luminescent organisms the light results from luminous slime secreted over parts of the body or thrown out as a glowing cloud about the animal, as in the case of the squid Heteroteuthis, which produces this secretion in a gland corresponding to the ink sac of better known squids. Many of the fishes and crustaceans possess very highly specialized luminous organs which in some cases are under nervous control. These organs are provided with light-producing cells, reflector, pigment layer, and lens. It has been observed (Macdonald, 1927) that six specimens of the crustacean Meganyctiphanes norvegica in a two-liter jar voluntarily emitted sufficient light for reading news print.
Only in relatively few instances can we perceive a usefulness of the light to the organism producing it. In dinoflagellates, bacteria, jellyfish, hydroids, and so forth, there appears to be no possible utility; but in the higher forms, particularly those with specialized light organs capable of flashing under nervous control and arranged in definite patterns and even specific colors, utility seems clear. Light can have meaning in these instances only if eyes are developed to see it. In this respect it is significant to note, as has been pointed out by Hjort (1912), that pelagic fishes living above 500 m have both well-developed eyes and luminous organs, while in the bathypelagic forms below 500 m both types of organs are diminished. An apparent anomaly then occurs at the bottom, where the eyes of the fishes are well developed but luminous organs are scarce
Confronted with the information which we now have on the distribution of organisms with eyes and on the highly complicated structures for light production (Brauer, 1906), we cannot but believe that luminosity is of specific use to many animals, although in many forms such as bacteria and protozoans its inception may have been only a secondary physiological manifestation.
The biochemical activities involved in bioluminescence are only in part understood. It is a highly efficient process since the light is practically without heat and is entirely within the spectral region of human vision, there being no rays of infrared or ultraviolet. The light is produced by oxidation of a substance, probably a simple protein known as luciferin, that is produced by the living cells but that may function even though separated from the cells. However, before light can be produced by union with oxygen it is necessary that another substance, luciferase, be present as a catalyst to accelerate the oxidation.
It has been suggested (Pierantoni, 1918) that wherever light is produced the actual agents are bacteria living symbiotically with the animals, but there is evidence that this is not universally true (Harvey, 1920).
Bioluminescence has been discussed at some length because, in commanding the attention of ecologists, morphologists, physiologists, bacteriologists, chemists, and physicists alike, it is representative of the problems of marine biology.
Vertical Migrations. Light is a significant factor in the behavior of animals both pelagic and littoral. It is therefore an especially important factor in the local or restricted (as opposed to geographic) distribution of animals. In the intertidal zone most animals are found in shaded situations, in crevices, or on the under sides of rocks or ledges. Much caution must be exercised, however, in attributing this type of distribution
The correlation of animal movements with light is best studied in the pelagic division of the ocean and we shall limit our discussion mainly to the animals of this environment. Numerous investigations of the vertical distribution of plankton organisms of the open sea have shown that many, if not most, of the pelagic forms make daily journeys from deeper water to the surface at the approach of darkness and begin returning to deeper water at or before the break of dawn. This rhythmic movement is known as diurnal migration. See Kikuchi (1930) for a review of various factors that have been considered as motivating forces. The range of depth covered and the time of movement vary with different species of animals. Those forms capable of moving vertically through several hundred meters of depth, whether in diurnal or irregular movements, are the eurybathic animals with a wide range of tolerance to rather rapid changes in pressure. Since the range of temperature may be considerable they may also be eurythermic.
A great amount of investigation has been carried on with reference particularly to diurnal migrations of pelagic copepods and chaetognaths, and these may be considered as typical of much of the plankton community and its movements.
The population does not accumulate and move in a compact body; rather, it shows a more or less continuous but uneven vertical distribution that sometimes extends over two or three hundred meters in depth. The greatest number of individuals will be found concentrated at a level where conditions are optimum with respect to light in relation to other factors. That portion of the population found at given distances below or above the optimum may consist of individuals of uniform age or sex (Russell, 1927, Gardiner, 1933, Nicholls, 1933). In one investigation (Nicholls, 1933) of a mixed population of Calanus finmarchicus, the adult females exhibited diurnal migrations to a greater degree than other members of the population. The following data will serve to illustrate the general nature of migrations in that species (fig. 232):
It will be seen that at 4:00 p.m. on January 24th the females were massed between 30 and 80 meters. The sun set at 4:27 p.m. and by 7:00 p.m. most of these females had moved into the upper 30 meters of water. Throughout
― 837 ―the investigation a small proportion remained in the deeper water irrespective of the migration of the bulk. By 10:00 p.m. the accumulation in the upper layers was more marked, but by 1:00 a.m. the descent had begun and females were evenly distributed from the surface down to 80 meters.At 4:00 a.m. they were concentrated between 30 and 60 meters with none at the surface. By 7:00 a.m. they were found between 30 and 80 meters. The sun rose at 8:37 a.m. and by 10:00 a.m. the upper layers were quite deserted; most were found between 60 and 80 meters, but a large proportion was distributed below 80 meters, and 4:00 p.m. on the 26th saw a return to the condition in which they were found at that time on 25th.
The more even distribution at 1:00 a.m. is interpreted as indicating the beginning of descent following complete removal of the stimulus of diffused light, but this scattering or descent apparently does not always occur until return of light at dawn (cf. Metridia, fig. 233; Clarke, 1933).
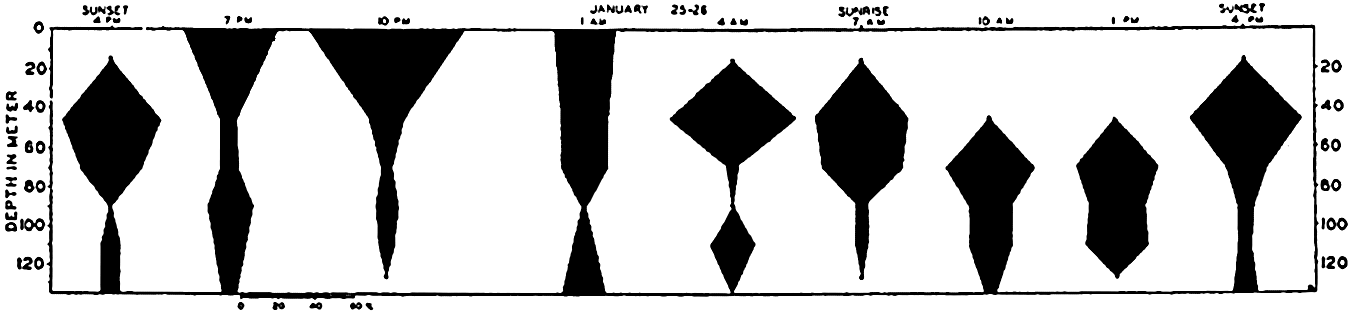
Diurnal migrations of adult female, Calanus finmarchicus (from Nicholls).
Not only do different species react differently, but different stages of development, and the sexes also, have their own characteristic behavior with respect to diurnal response associated with light. A further complication is encountered in that the degree of response is also seasonal, indicating changes in physiological state (Russell, 1928). Species of Acartia give some indication of a diurnal physiological state which causes migrations even in the absence of light (Esterly, 1917).
Since an animal is constantly subjected to various and simultaneous stimuli, some of fluctuating intensity, the nature of the movement will depend upon the combination at any one time, and it is not always clear which is the dominating stimulus.
In attempts to analyze these daily migratory habits several views have been expressed. It is agreed that light is the chief motivating factor, but we do not know whether it operates by virtue of its absolute magnitude of intensity or by change in intensity (Clarke, 1933, Johnson, 1938). Also it is not yet clear whether light evokes response directly or only indirectly through its modification of response to such factors as gravity, temperature, and food. The chief directional stimuli that plankton animals can receive are gravity and light, but the response to these may be modified by temperature, salinity, hunger for food, and so forth.
Gravity is an invariable factor constantly in operation, a force of momentous importance in the life of planktonic organisms. If it is not favorably overcome within water layers offering tolerable living conditions for the organisms it must result in their ultimate destruction in lower water layers or on the bottom. Some of the means employed to minimize this rate of sinking by wholly or relatively passive forms have been discussed elsewhere (p. 821). It must be emphasized that swimming upwards (negative geotropism) is of extreme importance, even to such weakly motile animals as copepods. But as observations have shown that plankton organisms have a twenty-four-hour cycle in which upward and downward migrations occur, it has been suggested that the intensity of light may be the agency affecting a change in the sign of geotropism (Esterly, 1917, 1919, Clarke, 1934). Thus in bright light the reaction to gravity is positive and the animals not only sink but may actually swim downward. In the comparative darkness of greater depths the effect of light becomes progressively less compelling and a threshold is finally reached, as is shown by a maximum density of animals at some depth. At midday this maximum will be found at its greatest depth. A reduction in light intensity with the approach of evening reverses the geotropism and the population spreads upward even to the surface. However, on approaching the surface, the population may sometimes encounter a sharp gradient of temperature, the thermocline, that may supply a sufficiently strong stimulus to dominate the field of stimuli and prevent further ascent toward the surface. Likewise a sharp thermocline limits the downward distribution of some surface forms. Observations in the Gulf of Maine show that the maximum number of Metridia lucens generally ascends to the thermocline, which has a gradient of about 7°C in 10 m, but does not pass through it (fig. 233); and Centropages typicus, which inhabits the warm-water stratum from 10 to 20 m, does not in general go below the thermocline into water of 8° to 5°C (Clarke, 1933).

Diurnal migrations of the adult female copepod, Metridia lucens. The changes in light intensity are indicated by lines representing the depth at which 1000 microwatts, 100 microwatts, and so forth, occurred (from Clarke).
Since most of the microplankton and diatoms upon which larger plankton forms subsist are produced in the euphotic layer, it has also been suggested that upward migrations may be made in search of food when light intensities are not so great as to be prohibitive (Worthington, 1931).
The phenomenon of diurnal migrations is not confined to animals living relatively near the surface. Sergastid prawns, for example, have their maximum abundance between 0 and 200 m at night and between 600 and 800 m during the day (Welsh, Chace, and Nunnemacher, 1937). We learned earlier that light is directional even at these depths and could conceivably be the directing force in very light-sensitive forms, as these must be. Dark-colored deep-water fishes were taken by the Michael Sars at the surface during the dark hours, whereas during the day they occurred only in the depths of much reduced light. The whole problem of vertical migrations is complex; and although we know that extensive vertical migrations occur with recurrent external conditions, yet they are variable in extent with respect to place and season, and need much additional investigation.
Other vertical migrations are discernible as general seasonal movements of certain populations. These movements are associated with critical periods, breeding for example, in the life cycle of the species involved rather than with light, and are best discussed elsewhere (p. 322). Since reactions related to spawning may be more or less spasmodic and contrary to the regularly observed reactions, so that extensive swarms may be found at the surface even during the daytime, they must be kept in mind in the interpretation of vertical movements.
Salinity
The character of the population of the sea is unique largely because of conditions inherent in the vast extent and depth of the marine environment, such as uniformity over vast areas; but the chief single factor which makes marine life in general what it is results from the salt content of the water.
It has already been mentioned that a close osmotic balance exists between sea water and the body fluids of the marine invertebrate organisms, and that this precludes the need of impervious teguments or special expenditure of energy in maintaining the body fluids at the proper concentration (chapter VIII). However, the marine invertebrates are usually poikilosmotic only within rather narrow limits and the concentration of salts is not the same everywhere in the sea, but in numerous instances the gradients are sharp and great fluctuations may occur in restricted coastal regions. Yet all conceivable habitats are occupied by a greater or smaller number of animals suited to the circumstances, even under very extreme fluctuations; this dispersion gives rise to a classification of animals as well as plants into two large groups, depending upon their tolerance to changes in salinity.
1. Stenohaline animals are those which are sensitive to relatively small changes in salinity. The animals of this group are especially characteristic of deep water and of the open sea, where over vast areas the
2. Euryhaline animals are those having a great degree of tolerance to wide ranges of salinity. They are naturally characteristic inhabitants of the coastal regions and of estuaries. However, owing to their tolerance, they are also frequently found far from shore as scattered specimens in the domain of more stenohaline forms. Apparently other factors related to the open sea prevent their successful propagation to a point of numerical dominance. The degree of euryhalinity varies greatly in different species. Intertidal animals, because of their periodic exposure to direct rains or runoff from land, are subject to considerable fluctuations in salinity, but when low tide is combined with unusually heavy rains the result may be lethal to much of the life on exposed flats and in small pools. Much damage may occur to oyster beds during excessively rainy seasons even though the adults may survive; major fluctuations in salinity in the Fal Estuary are held responsible for the failure of one fifth of the ripe females to spawn properly (Orton, 1937). Corals cannot live where rivers cause a persistent and great fall in salinity, although some reduction can be tolerated; the damaging effect of rivers may result in part from sedimentation. Vaughan (1919) reports that corals living in the tropics are tolerant to a 20 per cent reduction in the salinity of the water of theri normal environment. An extreme case of euryhalinity occurs in the harpacticoid copepod Tigriopus, which inhabits small pools usually reached only by the spray of breaking waves and therefore subject to great evaporation with attendant concentration of salts, or at other times to great dilution from rains. The calanoid Pseudodiaptomus euryhalinus is yet another copepod subjected to salinity ranges varying at least from 1.8 to 68.4 ‰. It normally inhabits small coastal lagoons or ponds along the southern California coast and from them it sometimes finds its way into the sea; these ponds are periodically diluted or concentrated (Johnson, 1939a). In these instances the animals have not sought the salinity in which they live by reaction to a gradient. They simply tolerate the salinity and their optimum concentration is not known. Completely tolerant forms are exemplified in the salmon and the eel, each of which spends a part of its life successively in marine and fresh-water environments.
In a study of the distribution and biology of animals we see that within the sea the salinity influences the character or type of animals that will be present in any region rather than the rate of reproduction or the total amount of animal organic material produced. However, the
Pelagic organisms, such as radiolarians that secrete silica, find their optimum living conditions in waters of somewhat lower salinity than that of the open oceans, and this provides a suggestion as to the condition of the Pre-Cambrian ocean when, judging from Pre-Cambrian rocks (Murray, 1895), radiolarians were apparently more abundant than now.
The relation between the salinity of the water and the prevailing faunas has been an invaluable aid to the study of paleogeography, and has led to an interpretation and understanding not only of the geographic arrangement of ancient seas, but also of the degree of salinity that must have prevailed when the fossilized animals inhabited the area. Conclusions can be drawn because many of these fossils are represented in present-day faunas by identical or closely related species in communities whose natural salinity demands can be ascertained. For example, it has been estimated by Ekman (1935) that during the Littorina stage the central and innermost parts of the Baltic Sea were about 5 ‰ more saline than at the present time. This is proved by a study of the then inner limits of distribution of certain molluscs. Littorina litorea, the common shore snail, formerly lived as far north as Sundsvall (62°20′N),
Size and Salinity. Among euryhaline animals, individuals living in reduced salinities frequently have a smaller maximum size than do those of the same species inhabiting more saline waters. This may indicate that the greater dilution is inimical and that the animals, although able to survive and perhaps even to reproduce, are living near the lower limit of their range of euryhalinity, and that only a slightly greater deviation from the optimum would cause death. On the other hand, the reduced size may result directly from failure of sufficient food supply of a type normally dependent upon higher salinity. This is to say that the organisms serving as the animal's food must be adapted to live in the same biotope or in one sufficiently near to enable it to drift in with water movements. Whatever the cause may be, it should be noted here that it is a strange and unexplained fact that with few exceptions marine animals from groups with fresh-water representatives are larger than the fresh-water relatives and usually the size difference is enormous. In planktonic forms this may be due in part to reduced viscosity of fresh water, but for the population as a whole the matter of food supply or rate of metabolism needs to be considered. Respiration is more difficult in fresh than in sea water. To this burden imposed by the environment is also added the necessity for fresh-water forms to expend special energy in overcoming the effects of the hypotonic surrounding medium and in maintaining a proper osmotic equilibrium. The fresh-water animals doubtless have suffered many vicissitudes not experienced in the more stable marine environment, but the effect of this is unknown.
To return again to the ecological significance of varying salinity in the sea, passive offshore plankton organisms, such as eggs and larvae having a specific gravity very near that of the water in which they float, are likely to lose their buoyancy, sink to the bottom, and be destroyed when they are swept by wind and currents into the less dense dilute waters near shore or at river outlets (Johnstone, 1908). In a study of the distribution of haddock eggs Walford (1938) concluded that the eggs tend to remain afloat in water of the same density as that in which they were fertilized. When forced into water of a different density, however, they are able at least within narrow limits to adopt a new specific gravity to conform with the water in which they drift. In the presence of
Much experimentation has been conducted relative to the specific biological effect or function of the various ions in sea water. It will not be possible here to give an adequate review of this important subject, which can be found discussed at length in special articles and texts in physiology and physiological chemistry. The function of salts in sea water is obviously not restricted to the maintenance of a favorable osmotic pressure with the body fluids, for many forms, notably fishes, are not isotonic with sea water in this respect. Waters of inland salt lakes may have a favorable osmotic pressure (resulting from concentration of various salts) for marine organisms, but other essential salts are wanting and the waters will therefore not support marine life. Even such forms as the shore isopod Ligia, which avoids being submerged in the sea, from whence it appears to be in process of migration, is unable to live unless its gills are moistened with sea water in order that it may acquire the essential salts and water useful in its respiration (Barnes, 1932).
Temperature
Temperature is a factor of prime importance in the marine environment because of its action (1) directly upon the physiological processes of the animals, especially upon the rate of metabolism and the reproductive cycle, and (2) indirectly through its influence on other environmental factors such as gases in solution, viscosity of the water, and density distribution with all its important hydrographic implications, discussed elsewhere.
An extensive literature has been built up pertaining to the effect of temperature on biological phenomena in the sea. This results first of all from the general recognition of the eminent importance of this factor and also from the fact that it is the most readily and accurately measured of the factors. This combination of circumstances may tend to give undue weight to the significance of temperature in relation to factors such as season, exchange or movement of water, and so forth, of which the temperature is only an index, and to factors such as density or viscosity which are functions of temperature (pp. 56 and 69). Nevertheless the abundant proof of its effects on life in the sea either directly or indirectly warrants a rather extensive review.
The range of temperature in the sea varies from about −3°C to 42°C, depending on season and locality, but in the open ocean the maximum temperature does not exceed 30°C. This range is small when
It should be remembered, however, that in the cold-blooded or poikilothermic animals living in an aquatic environment all changes of temperature of the external medium are accompanied (because of the high conductivity of water) by an immediate change in the internal temperature of the body. The regulatory mechanisms developed in many terrestrial forms are wanting and vital processes must consequently be affected accordingly. It is therefore not surprising that within the narrow temperature range found in the sea there are temperature barriers segregating faunas into rather well-defined geographical regions of submarine climatic conditions that are controlled not only by latitude but also by depth of water and general circulation.
Animals are commonly divided into two large groups with reference to their tolerance to temperature range, namely stenothermic and eurythermic animals. But, as with other classifications, there are many intergradations between the typically stenothermic and the typically eurythermic forms and the degree of tolerance may also vary with the stage in the life history of the individual. Nevertheless, the classification is sufficiently distinctive to characterize vast numbers of species and therefore faunas in general.
Within the temperature limits tolerated by an animal there are three fundamental temperatures. These are the optimum, the maximum, and the minimum. The optimum temperature for any given species is not readily defined, since it is evidently not the same for all stages and functions of life, as is indicated in reproductive cycles associated with season. The maximum and minimum refer to the upper and lower limits of thermal tolerance. They also vary with the stage of the life cycle and the past thermal history of the individual. It is generally thought that the optimum temperature lies nearer the maximum than the minimum, but recent studies on fishes indicate that this is not always so (Doudoroff, 1942).
In areas with severe winters the intertidal animals are likely to be relatively few in number owing to winter destruction by freezing or to abrasion of beaches by ice. Wholesale destruction sometimes occurs in milder regions during unusually cold winter periods. In a special investigation on the mortality among intertidal animals of Danish waters during an unusually severe and protracted winter period in which ice formed on the beach and sand froze to a depth of 15 cm, Blegvad (1929) found a very marked destruction of animals. The percentage of animals killed in the area studied is as follows: Mytilus edulis, 100 per cent; Littorina litorea, 100 per cent; Cardium edule, 80 per cent; Nereis diversicolor, 70 per cent; Arenicola marina, 95 per cent; Mya arenaria, 80 per cent; Macoma baltica, 33 per cent.
The optimum may be at the same or at a very different temperature for different species. Hence there are both cold-limited (phychrophile) and warmth-limited (thermophile) stenothermic forms, and as a result we find animals that are strictly endemic to cold- or warm-water areas of the ocean, as the case may be.
Geographic Distribution. It has been pointed out (Ekman, 1935) that early zoogeographers drew putative boundaries for littoral faunas based on climatic and hydrographic conditions. Rather little was known about the actual distribution limits of large numbers of species and faunal groups, but climatic boundaries had been discovered and the faunal areas were arranged according to these without their natural boundaries having been determined by empirical study. Zoogeographic boundaries must be determined in the final analysis by the actual distribution of the animals, but the marked importance of climate is shown by the fact that the early boundaries, drawn in the main speculatively, have been proved empirically to be well founded; which is to say that temperature is one of the most significant factors in the development of marine faunas.
Where temperature gradients are not well defined, the faunal zones do not have sharp boundaries either but merge one into the other with wide transition zones. The conditions in the Northeast Atlantic and the Northeast Pacific are considered especially illustrative of this. Along the east Atlantic coast, faunal boundaries are difficult to establish and a study of the isotherms of the North Atlantic reveals that the lines diverge toward the east and converge toward the west (charts II and III). For example, 36 per cent of the species of fishes found north of the Arctic Circle off Norway are found also in the Mediterranean. In the Northeast Pacific similar conditions are encountered, which are related to the divergence of isotherms on approaching the American continent. Here, however, the seasonal range in temperature is relatively small and upwelling along the coasts of North and South America keeps the coastal water cool to unusually low latitudes. The fauna of north temperate character has an extensive spread from the Bering Sea to Lower California, its closest affinities being with the Arctic rather than with the Tropical fauna. Among the arctic-temperate animals may be mentioned the genera Pandalus, Oregonia, Henricia. It must be noted also that in the east Atlantic boreal region the seasonal variations in water temperature are large in the coastal zone, having a range of 10° to 12°C off the coast of Norway and 10° to 15°C in the North Sea. These conditions tend to obliterate faunal boundaries for reasons discussed below.
Temperature and Reproduction. We have learned that there are many animals which are eurythermic while others are stenothermic, and that the stenothermic forms may be either warm stenothermic or cold stenothermic; that is, their optimum condition is at high or at low temperatures,
The very mixed fauna of the European boreal region is believed to be accounted for partly by these adjustments to suitable spawning season, for in these waters the seasonal range of temperature is sufficient to allow for northern and southern vegetative eurythermic forms to find periods suitable for stenothermic reproduction in the same area.
Results of examination of different species of oysters illustrate the variations in the critical spawning temperatures of marine animals. For both economic and scientific reasons, these animals have been studied in great detail by Trevor Kincaid, W. R. Coe, and others mentioned below, and the following minimum spawning temperatures have been established with considerable certainty (review by Hopkins, 1937):
Ostrea virginica 20°C (exceptionally at temperatures as low as 17°C or slightly less; Loosanoff, 1939) |
O. gigas 22–25°C (Galtsoff, 1930; Elsey, 1934) |
O. edulis 15–16°C (Orton, 1920) |
O. lurida 14–16°C (Hopkins, 1937) |
Although the above temperatures may be considered threshold temperatures, they can operate as determining factors only when other external and internal conditions are also within the favorable range. For example, the gonads must have reached the proper degree of development, which is itself a function of temperature and nourishment; the pH must be favorable, about 8.2 for Ostrea virginica (Prytherch, 1929); the presence of spermatozoa in the water may be necessary; and other uncertain factors also are involved (Galtsoff, 1930, 1938). The temperatures given above represent findings relative to the initiation of female spawning. Once begun it may continue at higher temperatures.
A good deal of evidence, both observational and experimental, shows that the distribution of many marine animals is dependent upon the above type of adjustments of critical reproductive periods within rather narrow temperature ranges. Orton (1920) concluded that:
A review of all the information collected bearing on the influence of temperature changes on breeding leads one to the conclusion that a temperature stimulus of some kind is the normal impulse for inducing sexual activity in marine animals assuming normal biological conditions. In marine invertebrates, at least, the impression is gathered that normal salinity variations within the habitat of the species have little effect on breeding. Since temperature is known to be of paramount importance in controlling distribution, it would seem that its influence on breeding is one of the ways in which the controlling effect is exerted.
Figure 234 and table 98, from an investigation by Runnström (1927), will serve to illustrate the relation between temperature and reproduction and larval survival of littoral marine fauna, of Arctic-boreal, boreal, and Mediterranean-boreal types of animals in boreal waters.
Vegetative distribution, especially of pelagic forms, may extend to areas beyond the limits set by the reproductive stenothermy; but such areas must be restocked regularly by adults or juveniles drifting in from favorable outside areas. This extended distribution is known as sterile distribution, and though the failure to reproduce in these extended areas may result from external environmental factors other than temperature, temperature seems nevertheless to be the major cause. It has been found, for instance, in the Bay of Fundy, that some species of fishes with floating eggs and such forms as the medusa Aglantha, the amphipod Parathemisto, the arrow worm Sagitta elegans, and still other forms either fail to reproduce at all or have very restricted success in reproduction although spawnings may occur (Huntsman and Reid, 1921, Fish and Johnson, 1937). The bulk of these animals must therefore be recruited seasonally by means of waters flowing in from the Gulf of Maine.
In the Gulf of Maine, Bigelow (1926) found that Sagitta serratodentata is a common constituent of the plankton. It thrives in these waters in the juvenile and adult stages, growing to a size greater than in its normal breeding habitat, but it is unable to breed in the Gulf because of the cold water. In the inner parts of the Gulf it succumbs during the winter and must be replenished each year by a new supply coming in with mixed water from the neighboring Gulf Stream. Its normal distribution is in waters above about 15°C.
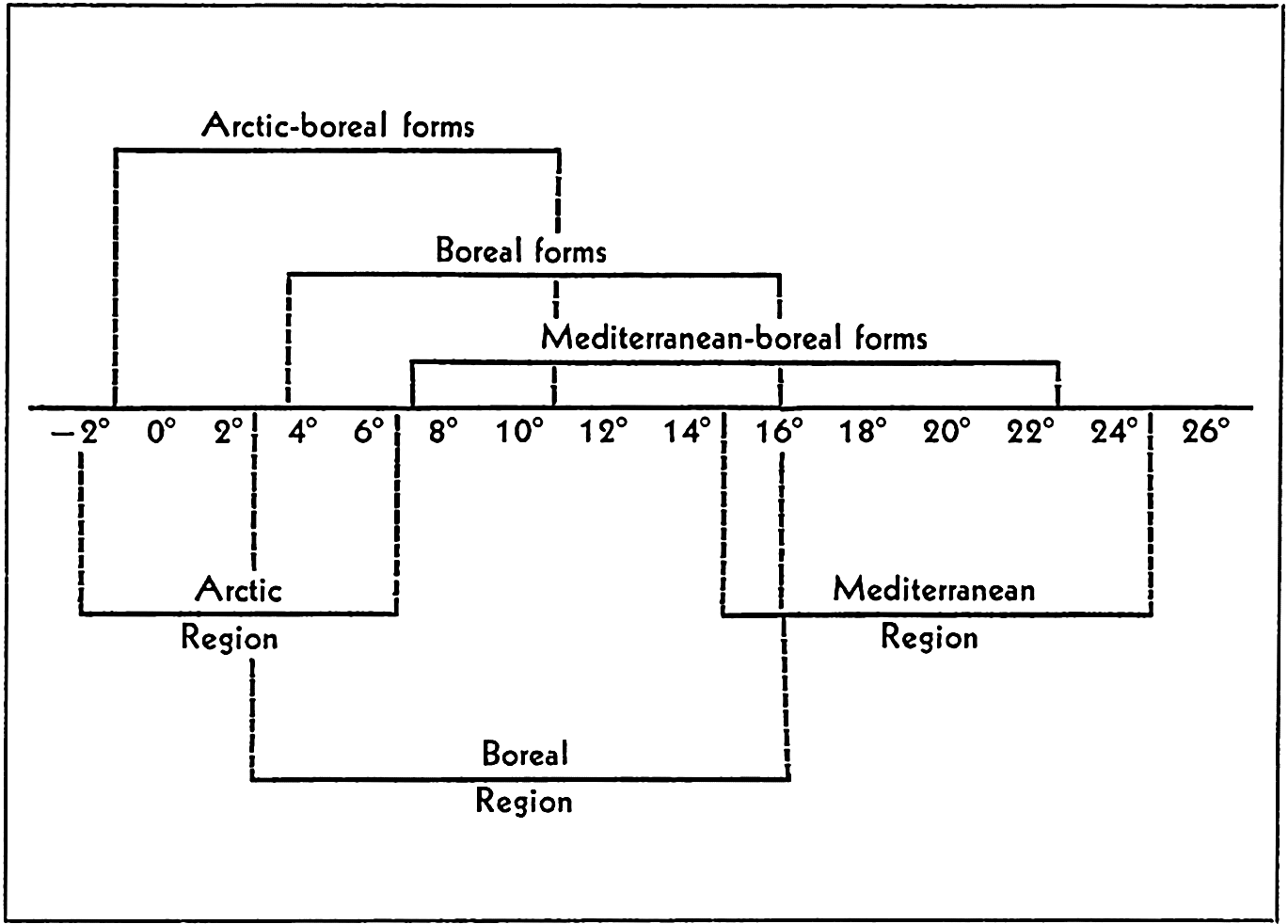
Temperature and reproduction. Lines above temperature series indicate the temperature range within which normal development takes place for each of the three faunal groups included. The lines below the temperature series give the temperature range for the regions indicated.
Fauna | Animal | Jan. | Feb. | Mar. | Apr. | May | June | July | Aug. | Sept. | Oct. | Nov. | Dec. |
---|---|---|---|---|---|---|---|---|---|---|---|---|---|
Arctic-boreal | Strongylocentrotus drobachiensis | + | + | + | |||||||||
Cucumaria frondosa | + | + | + | ||||||||||
Dentronotus frondosus. | + | + | |||||||||||
Boreal | Pleuronectes platessa. | + | + | + | |||||||||
Mytilus edulis. | + | + | + | + | |||||||||
Echinus esculentus. | + | + | + | + | + | ||||||||
Asterias rubens | + | + | + | + | |||||||||
Mediterraneanboreal | Psammechinus miliaris | + | + | + | + | + | |||||||
Echinocyamus pusillus | + | + | + | + | |||||||||
Echinocardium flavescens | + | + | + | + | + | ||||||||
Echinocardium cordatum | + | + | + | + | |||||||||
Ciona intestinalis | + | + | + | + | + | + |
The tropical and subtropical copepod Eucalanus elongatus is carried far north with the Gulf Stream, but only adult or submature specimens appear to survive in the gradually cooling waters approaching the higher latitudes (Farran, 1911, With, 1915).
One of the most striking instances of the effectiveness of temperature differences as a barrier to distribution of benthic animals in the open deep sea is shown by a study of the fauna on the two sides of the Wyville Thomson Ridge (Murray and Hjort, 1912). Over this ridge, covered by water to a depth of about 500 m and connecting the Shetland and Faeroe Islands, the Gulf Stream water flows into the cold Norwegian Sea (p. 652). At the top of the ridge temperatures on both sides are only moderately different, but bottom temperatures of 4°C are found at a depth of 1500 m on the south or Atlantic slope while on the northern slope in the Norwegian Sea the temperature is 4°C at about 600 m, 0°C at 850 m, and −0.41°C in the bottom waters. An analysis of the fauna of each side showed that only 48 species and varieties, or about 11 per cent of a total of 433 enumerated by Murray, are common to both sides.
Bipolarity. It has long been known that the faunas of the cold waters of the north and south latitudes contain many elements in common. The animals or animal groups that form these elements may be wanting in the intervening tropical region. A break in the continuity of distribution of a species or higher division is called discontinuous distribution; when it occurs in a meridional direction so that the animals involved are absent from the tropical belt, the phenomenon is known as bipolar distribution or bipolarity. According to the older concept, only the arctic and antarctic regions are involved, but more recent usage includes the temperate zones also, and bipolar animals need not necessarily be polar. This is bipolarity of relationship and may be defined as a bipolar distribution in which animals of higher latitudes are more closely related taxonomically to each other than to those of lower latitudes. There is also bipolarity of phenomena such as are associated with mass vernal production of diatoms, for example, or with production of great numbers of individuals but of relatively few species.
Associated with bipolarity of relationship is the phenomenon of tropical submergence. Stenothermic animals that require cold water can of course find this by seeking greater depths; if they are sufficiently euryhaline and eurybathic, northern littoral or surface pelagic animals may form a continuous distribution from high northern to high southern latitudes by living in deep water in that part of their meridional range
Examples of bipolarity are found in the gephyrean Priapulus caudatus of the Northern Hemisphere and a subspecies in the Southern Hemisphere. The pteropods Limacina helicina and Clione limacina of the north have related forms in the Southern Hemisphere. Other examples are the ascidian Didemnum albidum, and the shark Lamna cornubica. Bipolarity is not confined to species; the counterparts may be genera or families, in which cases bipolarity seems to be of older standing.
Since we are interested here mainly in showing the influence of temperature on distribution as illustrated by bipolarity, it is not proposed to discuss fully the means by which bipolarity was established. Two chief hypotheses have been advanced, the first holding that bipolar animals are relics of a previously cosmopolitan fauna, the tropical portion of which is now extinct; the second hypothesis holds that animals have migrated through cold deep water. A third hypothesis involves parallel development of the bipolar forms. It has been pointed out that the first two hypotheses do not conflict but are only different interpretations of the same idea, which we must accept under any circumstances if we consider the subject from the viewpoint of the history of development, to wit, that today's discontinuity emerged from yesterday's continuity. For one species the discontinuous distribution may have developed according to one hypothesis and for another species according to another; therefore each case must be studied independently.
Recently, through the purposeful efforts of man, a bipolarity has apparently been established in the Pacific salmon Oncorhynchus. The native distribution of this fish is confined mainly to the temperate waters of the North Pacific, but extends also into colder waters of the adjacent northern seas. Foreign transplantation of species of this genus has been successful in South Island, New Zealand, and at the southern tip of Chile. Many attempts to establish these migratory fish in the intervening areas of the tropical region between the native range and the new locations have met with failure. A study of the limiting environmental factors (Davidson and Hutchinson, 1938) shows that the temperature conditions at the newly established localities are comparable to those at the southern
The effect of temperature differences on animals influences the vertical as well as the horizontal distribution. This was illustrated by the tropical submergence of forms which, showing a continuous meridional distribution, inhabit both high southern and high northern latitudes.
In arctic waters, where the range of temperature between deep and shallow water is small compared with differences between shallow and deep water of lower latitudes, the demarcations between littoral and deep-water fauna are less clearly defined. Examples of this are the sea star Hymenaster pellucidus and the pycnogonid Nymphon robustum, each of which occurs in the Norwegian Sea at depths of 2000 m, while near Spitsbergen the former occurs at 27 m and the latter thrives at 6 m. It appears from these and many other instances that temperature and not depth is the controlling factor. In a study of recent foraminifera in ocean sediments extending from lagoonal conditions to a depth of 2540 m, Natland (1933) found that the vertical distribution of the animals was arranged in five zones, each with certain key species correlated with submarine temperatures. A complementary study showed the same type of zonation of the same species in fossil-bearing geological strata of outcrops and oil-well borings in the same general region, thus providing a means of arriving at the probable temperature and perhaps, therefore, also the depth of water and other conditions that prevailed when these strata were formed as sediments on the bottom of the sea. In other words, this zonation is illustrative of the fact that dissimilar fossil faunas may have been contemporaneous but may also have lived under different environmental conditions with respect to temperature.
Pelagic animals also show a degree of bathymetric stratification which may be associated with the temperature distribution in the sea. In this connection it is difficult, however, to isolate the effect of temperature from that of light or to find out whether the temperature exercises a direct or an indirect influence. Indirectly it may act through its influence on viscosity, or it may change the reactions of the animals to gravity or to light, that is, change the sign of tropism with respect to these factors. Even in the phenomenon of tropical submergence we must not fail to be aware of the fact that light, owing to the great intensity of the tropical sun, may possibly force some animals, especially pelagic animals, to seek greater depths.
In the discussion of diurnal migrations we noted that the sharp temperature gradient frequently encountered at the thermocline deters some of the deeper migrants from coming to the surface or some of those living normally near the surface from venturing below into markedly colder water (p. 838). Bigelow and Sears (1939) indicate that, aside from
Species of the fish genus Cyclothone descend to greater depths in southern than in northern waters. Among crustaceans the larger individuals of the pelagic prawn Acanthephyra purpurea occur at a depth of 500 to 750 m in the northern section traversed by the Michael Sars. In the lower latitudes these were found most abundant at 1000 m.
Metabolism. The optimum temperature for life activity is considered to be nearer the maximum than the minimum limit, for which reason a small rise in temperature from the optimum is more likely to be disastrous to cold-blooded animals than is a greater lowering (see p. 844). The latter will result in a marked slowing up of vital processes, but if not extreme may actually lead to an extension of the individual's life by inducing a period of quiescence when other factors, especially food supply, are at a minimum. This life extension may be illustrated by such forms as Calanus finmarchicus and C. hyperboreus, which during the winter months sink to, or seek, deeper water (200 to 300 m in some areas) and reduce their migratory activities (Gran, 1902, Sömme, 1934). Experimental tests have also indicated that Calanus grows and moults into successively older stages less rapidly at a temperature of 3°C than at temperatures of 6°C and 9°C, but survival is definitely better at the lowest temperature (Clarke and Bonnet, 1939).
The rate of metabolism (measured by oxygen consumption and production of carbon dioxide) of all poikilothermic organisms is very much increased with rise of temperature. According to van't Hoff's rule the increase is two to three times for each 10°C rise in temperature within favorable limits. Hence, it serves as one of the factors in the rapid and abundant spring production in boreal regions. During the winter when food supply is at a minimum in boreal waters, Calanus finmarchicus, for example, in copepodid stage V is found to sink to deep water, as already mentioned, where the temperatures are uniformly low and metabolism is accordingly slowed up. Here the animals may remain more or less quiescent until the return of spring, when they approach the surface, assume the adult state, and spawn in the upper water layers at a time when food has again become abundant. Among the coastal forms, the daphnids Podon and Evadne survive the low winter temperatures as resistant resting eggs. Rotifers and perhaps other coastal animals may do likewise, and are therefore able to appear suddenly in abundance in the spring plankton when vernal warming has taken place.
Numerous experiments have shown that oxygen consumption increases directly with rise in temperature. For a typical planktonic animal,
Calcium Precipitation. Temperature influences to a marked degree the rate at which calcium carbonate can be precipitated by animals in the formation of skeletal parts, shells, and spicules. The chemical reactions involved are not clearly understood, but they proceed more rapidly at high than at low temperatures; hence organisms that utilize calcium compounds in their supporting or protective skeletal structures are notably abundant in warm tropical waters, for in this environment shells can be grown faster and heavier than in the cold waters of higher latitudes or the deeper water layers where calcium precipitation is accomplished under great difficulty (Murray, 1895). Among the littoral life we need mention only (1) the marvelous coral faunas which have developed extensive reefs of great geologic importance and which have a wide north-south range in the western sections of the oceans but a narrower range in the eastern sections where the range of warm water is also narrowed, and (2) the beautiful and varied molluscan shells, including the giant clam Tridacna gigas, which may grow to a length of 1.5 m and a weight of 250 kg (551 lb). Conspicuous among the warm-water pelagic animals are the shelled foraminifera and pteropods.
Calcium-precipitating organisms are present in all seas, but their numbers are much reduced in the fauna of the cold polar seas and the shells of those present are relatively more weakly constructed. Some cold-water pteropods construct no shell, living naked, as some species of the beautiful and large Clione. Limacina helicina of Arctic waters does possess a shell but the animal is reduced in size and its shell is very thin. Among the foraminifera, a group apparently very sensitive to temperature conditions, the calcareous-shelled species are replaced in cold northern waters by the arenaceous types, which build shells of sand, fragments of shells, spicules, and so forth, cemented together with noncalcareous cement. Arenaceous types are also characteristic of deep-water foraminifera. In very deep water, therefore water of low temperatures, the skeletons of benthic animals are notably thin and fragile, as exemplified
This restricted production of calcareous structures in deep-sea animals can hardly result from a lack of lime for many poorly calcified forms are found where the bottom deposits consist of globigerina ooze which has resulted from precipitation of pelagic foraminifera living in the upper layers. Low temperature is apparently a major cause of the limited production of calcareous structures although the great hydrostatic pressure in deep water may be of importance because it is believed to increase the solubility of calcium carbonate (Buch and Gripenberg, 1932). The absence of violent water movement or even moderately fast flow in the deep sea may also be responsible for the small deposition of calcium by sessile organisms, and the absence of strong motion makes it possible, on the other hand, for many of the fragile-shelled and weak organisms to survive where otherwise they could not exist.
The effect of temperature on biological deposition of calcium carbonate provides a key to the interpretation of temperature conditions and water depths that must have prevailed at periods when certain geological strata of marine origin were laid down in the distant past. The present slight deposition of lime in cold polar regions, as contrasted with what must have occurred when ancient coral reefs were constructed there in Paleozoic times, leads to the theory that these ancient seas must have had a temperature of about 15° to 18°C (Murray, 1895).
Coral-reef formation is a most striking example of marine biological activity, which has attracted wide attention of both laymen and scientists. The coral reefs do not result from spectacular outbursts of activity such as occur among pelagic forms, but rather from the persistent accumulation of calcareous deposits which remain long after the cessation of the biological activity which produced them.
These great structures are outstanding features of the tropical seas. However, since the process of reef formation is a result of biological precipitation of calcium from sea water by corals, the rate of deposition is thermally controlled, as with other organisms. The inequalities of temperature distribution along the continental margins of the tropical
The organisms entering into reef formation are of several types, including chiefly the stony or madreporarian corals, but associated with these are also a number of other calcium-depositing coelenterates such as the Millepora. Very important are the foraminifera and a number of calcareous coralline algae known as the nullipores, of which the red Lithothamneon and the green alga Halimeda are examples. The nullipores in particular contribute vastly to reef formation. In some reefs corals play only a subordinate role.
The reef-producing corals can flourish only in water above about 20°C and are therefore confined to the shallow water of tropical seas. Although conditions are unfavorable for growth below a depth of 50 to 60 m, the slopes of reefs are known to extend to great depths. Also, through examination of material brought up from deep borings (340 m, 1114 ft) within the reefs it is shown that the structure of coral reefs extends to depths far greater than are tolerated by the living animals or plants that enter into the formation of the reefs. Obviously, if the coral fragments occurring at the base of the reefs were laid down in situ, the water level must have been at one time near the level of the base. This implies that the sea bottom on which the reef rests was once at a higher level, or that the sea surface was lower than we now know it.
The subject of reef formation from geological and biological viewpoints has been dealt with in an extensive literature to which the interested reader is referred (see especially Davis, 1928, and Gardiner, 1931). For our purpose it will suffice to state here that the theories which have been advanced largely involve changes in sea level with respect to the substratum on which the coral-building organisms initiated their growth. Some theories assume a sinking of the earth's crust, others a rise in sea level, or a combination of both. Without entering into details on these theories, we shall point out that here is illustrated a problem of the sea the interpretation of which is directly dependent upon an understanding of the organisms involved, for any theory advanced must take into account the biological requirements of the reef-building organisms, which comprise warm, relatively shallow, clear, saline water.
Temperature and Size. There is much evidence, from direct observation of populations obtained from various waters, to indicate that frequently cold-water animals grow to a larger size than do similar animals in warm water. A few examples will suffice to illustrate this point.
The warm-water epiplankton populations of copepods, the most characteristic of plankton animals, stand in striking contrast to the cold-water populations because of the apparently greater success of the larger species in the cold waters. A fair number of small species of such genera as Oithona, Oncea, Pseudocalanus, and others, of size ranging from about 0.5 to 1.5 mm, do live in cold northern waters and, conversely, a number of large forms, for instance, Eucalanus elongatus (8.0 mm) and Rhincalanus cornutus (3.4 mm) are found in warm waters; however, the warm-water populations are especially characterized by a large number of small species whereas the populations of arctic and boreal waters are mainly composed of great numbers of individuals of a few species, for example, Calanus hyperboreus (10 mm), C. finmarchicus (5.4 mm), C. cristatus (9.3 mm), Eucalanus bungii (8.0 mm). The deep cold waters of lower latitudes are also populated by large forms comparable in size to northern species. The numbers of individuals are, however, relatively fewer.
This tendency to larger size in colder waters is noticeable in related species and even in individuals of the same species. Jespersen (1939) reports that Calanus finmarchicus in Greenland waters forms two size groups, the larger being found particularly in waters of low temperature and the smaller-sized population in warmer surface layers of pronouncedly Atlantic waters. Steuer (1933) found a three-modal curve for body size of three varieties of Pleuromamma taken in the Benguela stream. A dwarf variety (P. minima) occurred in the uppermost, warmest water, a medium-sized race (P. piseki) in the middle layer, and a giant race (P. maxima) in the lower and coldest layer at about 600 m. Steuer has shown also that individuals of the copepods Acartia negligens and A. danae become smaller in transition from the Canary Current to the warm Guinea Stream. Similarly A. danae is larger in the South Equatorial Current, which is mixed with cold water of the Benguela Current.
Some radiolaria, especially the Challengeridae, have been shown to increase in size progressively with depth. In the genus Challengeria eight species can be grouped into three size categories: 0.11 to 0.16 mm, three species; 0.215 to 0.28 mm, three species; and 0.35 to 0.58 mm, two species. These categories correspond to depths of 50 to 400 m, 400 to 1500 m, and 1500 to 5000 m, respectively. Thermal influence on the size of pelagic protozoans is well illustrated also by the ciliates Tintinnoinea collected by the Agassiz Expedition. An analysis of the dimensions of loricae of 1000 individuals of a single species (Tintinnus tenue) of these animals showed that those taken in the Peruvian Current stations with surface temperatures of 19° to 23°C, were predominantly larger than those taken elsewhere at stations with temperatures of 24° to 28°C (Kofoid, 1930).
The effect of this factor on size is indicated also in the plankton fishes. Investigations of the Michael Sars showed that the small deep-sea fishes
Two explanations have been given to account for the increased size of cold-water planktonic forms.
(1) The increased density and viscosity of cold waters enables large forms to keep afloat more successfully in cold than in warm water (p. 822). The matter of overcoming the constant action of gravity is of paramount importance to weakly swimming animals like the smaller crustaceans, as well as to the passively floating radiolaria and many other forms. A size commensurate with viscosity and swimming power is one of the means of overcoming this ever-present tendency to sink. Just how important viscosity may be for delicately adjusted forms is suggested by the fact that the value of the viscosity at 50 m in the Norwegian Sea corresponds to that at 800 m farther south in the Atlantic (Murray and Hjort, 1912).
(2) Lowered temperatures lengthen the time required for poikilothermic animals to reach sexual maturity. Hence, in cold-water forms the delay permits a longer growing period with resultant larger size at maturity. It has been shown that the oxygen consumption of certain nonlocomotory warm-water benthic species is higher than that of related cold-water species with which they were experimentally compared, and this difference in metabolism may have a bearing on the question (Fox, 1936).
The more rapid attainment of sexual maturity in warm-water animals has certain important implications in that it permits a more rapid succession of generations in waters of tropical and subtropical regions. To illustrate this we may refer again to the oyster, Ostrea virginica. In a study of the sexual phases of large numbers of these bivalve molluscs, Coe (1938) found that
From Cape Cod to the Chesapeake Bay, most of the young oysters may be expected to spawn at the age of one year. North of Cape Cod the first spawning is stated to occur more often at the end of the second year, as is the case with some individuals in certain years south of Cape Cod. On the coast of North Carolina and in the Gulf of Mexico, well-nourished individuals of the early set spawn toward the end of their first summer, when only three to four months of age, while those of the later set do not become mature until the following spring.
An increased rate of reproduction is also common in other animals. The more rapid turnover of generations and the more nearly optimum living conditions of lower latitudes, with relative absence of drastic seasonal changes associated with temperature conditions, apparently lead to production of a greater number of species of most animal groups and to
Ocean Currents
If the waters of the oceans were not kept in vigorous circulation by the various forces discussed elsewhere, they could support only a small fraction of the marine life that actually now exists. First of all, the amount of primary food produced by plants would be so curtailed by exhaustion of the essential nutrients in the euphotic zone that only a few animals could find nourishment. The slow diffusion of gases and toxic wastes that could take place would set shallow vertical limits of distribution to all forms of life except certain anaerobic bacteria. The northern and southern boundaries of faunal zones dependent on temperature or light would coincide with parallels of latitude.
But the ocean waters are not stagnant. Vertical circulation extending to great depths occurs, and greater or smaller masses of water flow continuously in systems extending in diverse directions over vast areas, irrespective of latitude. Far to the north along western continental shores in the Northern Hemisphere the currents convey heat that has been stored in the waters during their passage through warm, tropical regions. Similarly, along the eastern continental shores cold water masses are carried to the south by currents of arctic or subarctic origin, causing isotherms to streak obliquely across the oceans in a general northeasterly direction. Systems of similar character and magnitude exist in the Southern Hemisphere (Charts II and III).
Water movements provide a means of transportation for organisms which, when combined with temperature and other factors, may control the limits of propagative and sterile distribution of both pelagic and benthic life. The character of the fauna and flora is thus governed by the nature of the currents—namely, their origin, direction of flow, magnitude, coldness or warmth, degree of salinity or density, and their character as regards other attributes such as relative richness in nutrients.
We have just reviewed some of the influences of temperature on animal life. Some of these influences are inseparable from ocean currents, for temperature is usually an index of flow. With this in mind, we shall now endeavor to examine more closely some features wherein water movements are of far-reaching biological significance.
Significance of Currents in Dispersal and Maintenance of Populations. Perhaps the most obvious importance of currents on marine life results from its direct influence on dispersal. It has already been pointed out (p. 315) that nearly all benthic animals in the littoral zone possess free-swimming or floating eggs and larvae. The significance attached to this type of life history in any given species must be that it provides a means of taking advantage of water movements to bring
about and maintain as wide distribution as possible, so that all environmental facies favorable to the life requirements of the adult both for spawning and vegetative growth can be inhabited. During this dispersal, countless thousands of larvae are carried into areas where they are unable to live or where at desertion of the pelagic life the sessile stages find conditions unsuitable with respect to depth of water, substratum, chemical-physical character of the water, or such other factors as food and enemies. This, however, is the price paid for the widest possible distribution.
Vast numbers of young are accordingly produced by each individual in order to overcome the hazards of the temporary pelagic existence (see p. 316). By far the largest number of larvae of benthic forms are eaten by predacious animals or succumb as a result of settling in inimical environments at the time of metamorphosis and desertion of the pelagic habit. That great numbers, however, do find favorable settlement is witnessed by extensive populations of benthic animals. Certain areas may be quite suitable to maintain a population but this population may be unable to restock to any appreciable extent from its own spawning, owing to the prevailing currents carrying the eggs and larvae completely away from the area and into other situations where settlement may or may not occur. These areas cannot be considered as areas of sterile distribution for reproduction does take place and larvae can survive, but because of circumstances associated with currents the areas must be regularly restocked by incoming larvae from elsewhere. Most benthic populations, perhaps, experience to some degree such renewal of stock by larvae produced elsewhere, and in turn they contribute their own larvae to restock other areas. Oscillating currents related to tides within a bay or fjord function to reduce loss of larvae and to maintain a degree of independence for its population.
When the larvae of benthic forms have survived to settle in a favorable habitat, the new stage of life is still dependent upon currents to maintain tolerable living conditions with respect to aeration, dispersal of toxic wastes, and supply of planktonic food. To coastal animal communities where the temporary plankton element is conspicuous, coastal circulation resulting from more or less local conditions such as tides and winds is of especial importance; but we shall see that larger and more permanent current systems also affect the lives of members of the temporary plankton and their adult stages.
As illustrative of the role of currents in the life of coastal benthic animals we refer to the study of Emerita analoga (Johnson, 1939b), the sand crab which in the benthic state inhabits sandy beaches in the intertidal zone. The female incubates the eggs, which remain attached to body appendages until the first zoeal larval stage. Then the larvae are freed to seek food and to face the regular hazards of enemies and possibly
The distance to which these or other temporary larvae can be transported depends of course upon the speed of the currents, the adaptability of the larvae, and especially upon the normal length of pelagic larval existence. In Emerita the larval stage is of long duration, being a matter of about four to five months and resulting in transport seaward at least 200 miles and doubtless much greater distances in coastwise currents. In the oyster the larval stage is shorter, being only about two to four weeks, depending upon temperatures. Yet oysters are known to extend their range of distribution at least 30 miles in one season through drift of the pelagic larval stage (Elsey and Quayle, 1939).
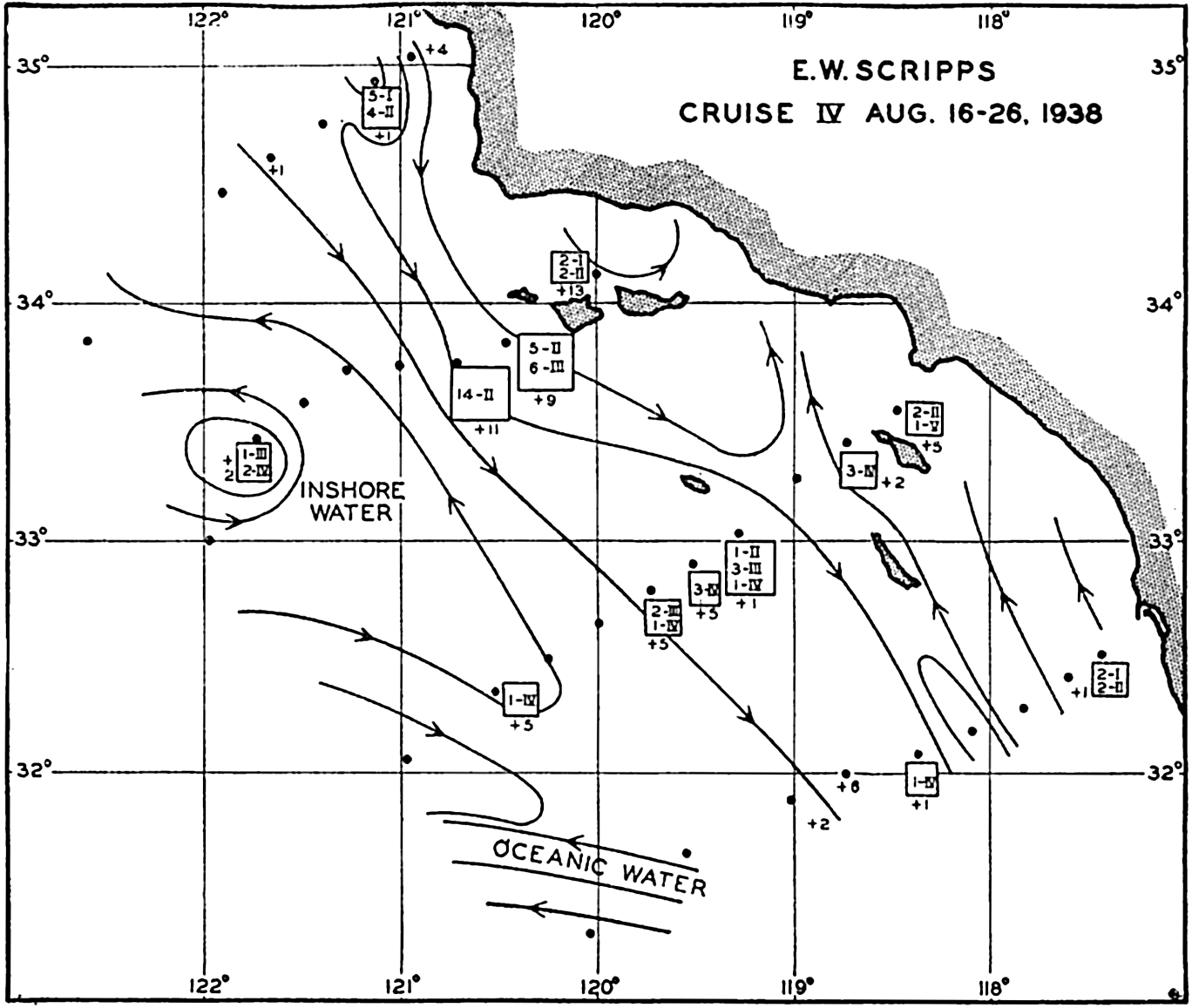
The dispersal of larval stages of Emerita analoga and other littoral crustacean larvae. Figures within the squares indicate the number and zoeal stages of Emerita; the symbol + followed by a number indicates the number of unidentified crab larvae. The character of the currents is indicated by the lines with arrows. (From Johnson.)
The great stretch of open water between the west coast of Central America and the easternmost Polynesian Islands is considered an effective
The depth at which passive eggs of the temporary plankton are carried by water currents depends to a great extent on their specific gravity in relation to that of the waters wherein they were spawned or to which they may rise (Walford, 1938). It was found (Thompson and Van Cleve, 1936) that halibut eggs occur in the denser waters some distance offshore at the outer edge of the spawning banks in depths of 100 to 200 m, though occasionally at only 40 m and at other times as deep as 935 m. The eggs were completely absent in shallower net hauls near the surface in waters with a low salinity and low specific gravity. From these facts it appears that they drift with the slowly moving deep currents. The newly hatched halibut larvae are found outside the continental shelf and at depths over 200 m. As the larvae develop, however, they rise to the upper layers until at the age of three to five months all of them are found at depths of 100 m or less. With the rise to the surface they are carried by inshore currents into shallow Alaskan bays, where they are found on the bottom. The cycle requires about six or seven months for completion. In the southern range of the northern halibut the powers of recuperation of the overfished stock are apparently less than in the stock of the Alaskan area, owing to a more unfavorable dispersal of the pelagic eggs by offshore or southward-moving currents in the southern area (Thompson, 1936).
Perhaps the best known example of transoceanic transportation of organisms is found in the investigations of Johannes Schmidt (1925a), dealing with the life history of the European eel Anguilla vulgaris. Full-grown and submature eels inhabit fresh-water streams and lakes, but upon approach of complete sexual maturity in autumn they desert these waters and disappear into the sea to spawn. The destination of the eels during these spawning journeys was for centuries one of the deepest mysteries of the sea. The young, recently metamorphosed eels, known as elvers, (about 6 or 7 cm long) have long been known to occur in the European coastal waters where in spring they enter fresh water in great numbers, but it was not known where in the sea they had originated. Strange theories were advanced; for example, Pliny and Aristotle believed they arose from mud of the sea bottom. Only in the last few decades have these mysteries been solved. Not until 1896 was it shown (Grassi, 1896) that a strange ribbonlike little fish, considered since 1856 as a separate species called Leptocephalus brevirostris, was in reality an eel developmental stage which through metamorphosis becomes the elver.
With this information at hand Johannes Schmidt in 1904 began investigations which extended over many years, involving examination
The American eel Anguilla rostrata breeds in the same or adjacent waters. Its life history requires only two years of larval life, for although the bulk of larvae migrate for a period in the same current as the European larvae, upon nearing the North American coast they become segregated from the European larvae, which are destined to continue slowly onward to the east.
The animals belonging to the permanent plankton may also be transported by currents over great distances, and this is particularly true with respect to the main oceanic currents. If the courses be sufficiently long or circular, the animals will successfully propagate while en route. Successful propagation is indicated when all stages of development of a given species are found in the transporting waters. Usually, however, the waters change their character as regards temperature, salinity, and so forth, or they may merge with other waters and lose their identity entirely or in part. In this case many of the animals that are being transported may die. Some of the hardier species frequently survive for some time and may even spawn, but the young are unable to withstand the environment unnatural to them (see p. 863). In this event the plankton catches will show only adult or submature stages, sometimes eggs and a few early stages, but few if any of the intermediate ones. Restocking must then take place regularly or periodically in successive invasions from the outside. The presence of these exotic animals is living evidence of the source of the water, provided the normal habitat of the species is known. Gulf Stream species are classical examples of planktonic animals that appear as visitors in localities where they do not normally breed. Illustrative of this are certain salps and the tropical copepod Rhincalanus nasutus occurring off the coast of Norway. Other examples are found in the northern penetration of R. nasutus and also
On the west Atlantic coast a number of Gulf Stream forms are swept by offshoots of the main current into the Gulf of Maine. They may survive there for varying periods of time, although the temperature and salinity are much reduced by mixture with coastal waters. Bigelow (1926), by a study of plankton catches, has determined the point of entrance of these immigrant forms and has traced their course after entering the Gulf and mingling with the endemic plankton community. They enter the Gulf at three levels and show varying degrees of success in establishing themselves, depending upon their species and adaptability. It is found, for example, that Sagitta serratodentata is a very hardy warmwater visitor that survives long enough to complete a good portion of the large counterclockwise gyral within the Gulf, and it may even grow to a larger size than in its native Gulf Stream habitat, although it cannot reproduce with sufficient success to establish itself as endemic. Adults may live for a time in waters with temperatures as low even as 3.9°C but do not survive the winter in the invaded area. Redfield and Beale (1940) have verified Bigelow's conclusion (1926) that this species does not reproduce in the Gulf of Maine. Because of this sterile distribution the animals are known as terminal immigrants. Their periodic occurrence depends upon periodic changes in the flow of outside water into the area. Sagitta maxima enters the Gulf in the deep layers and becomes dispersed in the deeper part where salinity is highest. Rhincalanus cornutus and R. nasutus are also stray tropical and oceanic visitors in the Gulf.
Arctic forms are also found in the Gulf of Maine, giving evidence of the penetration of the southward-flowing cold Nova Scotian water past Cape Sable into the Gulf. Among these cold-water strays are included Calanus hyperboreus, the pteropod Limacina helicina, and the appendicularian Oikopleura vanhoffeni.
The pattern of horizontal flow is a determining factor not only in the widespread dispersal of exotic plankton elements from their centers of production, but also in maintaining an endemic population. The effect of oscillating tidal currents with a minimum residual flow was mentioned above as contributing to the maintenance of an endemic stock in restricted areas. Many partially landlocked bays maintain a characteristic population through such action. The semiclosed lochs of the Clyde Sea area, for example, have proved favorable regions for scientific study of local population cycles without too great an exchange with biological elements produced elsewhere and carried into the lochs (p. 903). But certain types of nontidal current systems are also effective in supporting a self-sustaining population of specific forms. Such are the eddies of
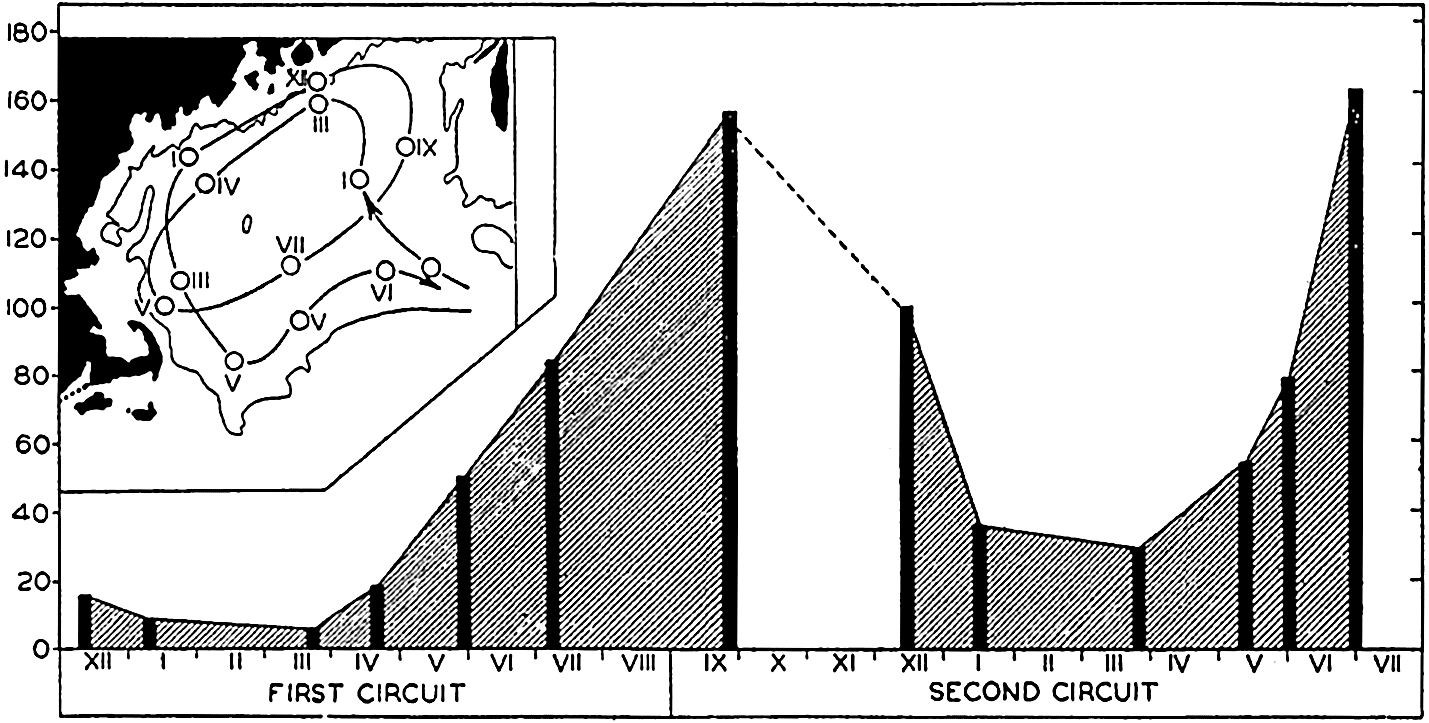
The growth of population in a mass of water assumed to move along the course indicated in inset. Ordinate: volume of zooplankton in cubic centimeters per square meter of sea surface; abscissa, time in months. The black bars indicate the volumes caught at the selected stations. The position of these stations and the month of collection are indicated on the inserted chart. (From Redfield.)
Damas (1905) and Sömme (1934) have discussed the importance of such currents in maintaining a breeding stock for pelagic populations in the Norwegian Sea. The Gulf of Maine on the American coast offers an excellent example of the biological effect of this type of circulation. Redfield (1941), carrying forward the earlier analyses of Bigelow and of Huntsman, concludes that the great cyclonic eddy of the basin of the Gulf is a vital factor in maintaining sufficient breeding stock to perpetuate an endemic calanoid population (Calanus finmarchicus and related forms) in that area and also to contribute to other neighboring waters. The regular recurrence of a closed eddy during the summer provides a tolerably uniform environment permitting the endemic species to complete their whole life cycle from egg to spawning adult while being swept involuntarily along in the moving mass of surface water wherein the bulk of the animals occur. Figure 236, from Redfield, shows how the shift of population center was followed in its drift around the Gulf. In chapter IX we learned that the rate of breeding of C. finmarchicus permits of two or three generations in a year. The rate of flow of water within the Gulf is about seven miles per day and requires about three months to make the complete circuit, thus allowing considerable time for development. In early winter there is an indraft of relatively barren water from the northeast, and also during this time the center of the endemic population is being forced gradually to the southwest, but in
A cyclic circulation resulting in a flow of water around an island may also lead to maintenance of an endemic stock. A good example depicting this is the investigation of the drift of eggs and larvae of gadoid fishes around Iceland (Schmidt, 1909). Hydrographic investigations show a clockwise current which flows around the island at a speed just sufficient to transport the developing eggs and larvae passively from the spawning ground, located on the south and west coasts, to the north and east coasts where the advanced larvae seek the bottom to continue growing after their coastwise journey of several hundred miles in the plankton. The motility of the older fish is also a factor in maintaining the local stock, but any circuit of this nature must be a particularly significant factor in maintaining an endemic population of benthic life and of the general plankton as well.
Finally, the biological implications resulting from a difference in rate of flow of surface and subsurface water masses in any given area should be mentioned. Planktonic animals that inhabit deep water and that perform no vertical migrations must cross into other water masses less frequently than those making vertical diurnal migrations. Periodically the latter will become segregated horizontally from the former when their journey takes them into waters with different rates of flow. Different stages of development or even the sexes may experience some degree of segregation from this cause, since it is known that the adults and juveniles, as well as the sexes of some species, do not all migrate at the same rate (p. 837). Hardy (1935) has shown how vertical migrations and the dynamics of distribution may function to segregate phytoplankton and zooplankton swarms (p. 900).
Certain areas of slowly moving water at depths of 300 m or more are held responsible for the maintenance of a stationary winter population of Calanus finmarchicus in the Norwegian Sea (Sömme, 1934). If these animals were to winter in the surface waters instead of descending to greater depths, they would become dispersed by the more rapid surface currents and thus dilute the breeding stock for spring production.
Indicator Species. From what has been said of the far-reaching biological influences of water movements, it becomes obvious that the intelligent interpretations in any marine ecological investigation must be based in part on data which provide a reliable picture of ocean currents both locally and in general. Descriptions of current systems and discussions of methods for observing or computing currents are given elsewhere,
We have seen that many animals are carried passively by currents into areas other than those in which they normally live and breed. If now we consider the problem conversely, these exotic forms whose developmental history, habits, and source are known become invaluable indicators of the source of the currents or water masses in which they are found.
A review of the voluminous literature bearing upon this subject is best obtained in recent special publications (Russell 1935a, 1935b, 1939). As examples of indicators we need here only refer back to the forms used in illustrating the dispersal of animals by currents. It must be born in mind that for most reliable use of indicator species the following pre-requisites are necessary:
Exact identification of the species or varieties of organisms involved must be possible.
Their propagative and sterile distribution must be satisfactorily known.
The morphological stages of development in the life history must be known for the species or must be deducible from what is known of the group to which they belong. In this connection the time and depth at which spawning occurs and the duration of the various stages are important. Passive eggs and larvae may be adjusted in specific gravity to heavy or light water layers and therefore transported mainly in layers of corresponding density (p. 861).
It should be known to what extent ecological adjustment can be made by migrations into deeper or shallower water in the extended geographical distribution. For example, Calanus hyperboreus and Parathemisto oblivia, arctic species, may live in deep cold water in lower latitudes and by vertical migration may appear also in surface layers (Gran, 1902).
A clear distinction must be made between animals brought in from outside geographical areas and those of biological groups that develop locally when conditions simulate those of the outside geographical area, especially with respect to temperature (see p. 793).
Associations of organisms transported from a common center of distribution are of more value than occasional strays. Such associations may be expected to be found especially among the euryhaline-stenohaline neritic forms occurring in offshore situations, indicating seaward drift of coastal waters. Pelagic larvae of intertidal benthic species may be of special value if the duration of the various larval stages is sufficiently well known to supply information not only on distance of drift but also
― 867 ―on the time involved (fig. 235). These larvae thus serve as myriads of tiny drift bottles released along the coast.
Species Development. Indirectly, currents must have played an important role in the development of species. Through the ages, the currents have persisted in the dispersal of marine animals and many euryhaline and eurythermic species have become cosmopolitan. However, distributional limits are usually much more narrow for most animals since the scattering effect of currents must constantly work in opposition to the limiting effects of other factors and in particular to those of temperature, salinity, and light. It appears that the persistent pressing by currents of both the holo- and meroplanktonic forms into regions deviating from the optimal living conditions has had profound effects on the character of the fauna established in the more adverse regions. The less tolerant species are eliminated and certain genetic strains adapted to the more selective conditions are developed. It is generally believed that species-producing centers occur in the temperate or warm stable waters of the tropical belt. Strongly indicative of this is the correlation between temperature and the number of species of Tintinnoinea (Kofoid, 1930). Of the 705 known species of the suborder, 8 are restricted to fresh water, 59 to the Arctic seas, 42 to the Antarctic, and 515 to the warm temperate and tropical oceans. Though the polar faunas are not so fully known as the tropical, the ratio of cold- to warm-water species appears to be one to five.
Not merely species but many genera and even higher systematic groups are confined entirely, or nearly so, to the tropical region (Ekman, 1935). From these rich centers temperate and cold-water species have seemingly evolved through the process of selection at border regions where conditions become farther and farther removed from the optimum for stenothermic warm-water forms. The more rigorous living conditions of bordering areas have allowed only relatively few species to become established compared to the abundant fauna of tropical waters. This relation is brought out also for certain of the higher pelagic animals in table 99, adapted with modifications from a summary of the better-known holoplanktonic groups which was compiled from various specialists by Russell (1935b). The numbers given are not to be considered final, for it is certain that many species have not yet been recorded or are not included, but they are sufficiently complete to illustrate the preponderance of species in warm areas and to indicate other relative features to which reference will be made later.
The greater number of planktonic species in the antarctic region than in the arctic may be associated with the far greater area of confluence between the warm and cold waters in the Southern Hemisphere but, in addition, the general oceanic currents of the Atlantic appear to play a part, especially for the cold-water copepods (Russell, 1935b).
Cold-water species evolving outward from warm-water surface species may do so by dispersal either in a northerly or southerly direction, and also by submergence into the deep cold water of the tropics where deepwater genera and species are formed. The deep-water current system in the Atlantic (p. 747) favors the carrying of these deep-water forms, as well as those of the Arctic, toward the antarctic surface region (p. 620). That the antarctic and subantarctic copepod population has a closer connection with deep-sea genera is shown by the fact that in the Antarctic and Subantarctic 18 species belong to deep-sea genera (that is, genera in which 50 per cent or more of the species in a genus may be regarded as belonging to the deep sea), as opposed to only 3 species belonging to deep-sea genera in the Arctic and in boreal waters. Species that are bipolar-epiplanktonic (p. 850) are representatives mainly of surface genera (that is, genera in which less than 50 per cent of the species in a genus can be regarded as deep-sea species). Judged by these standards, 5 species of bipolar-epiplanktonic copepods are among surface genera and only 1 species from deep-sea genera. The extent to which bipolar-epiplanktonic species can develop apparently depends largely on the ability of northern cold-water species to withstand tropical submergence.
Animals | Arctic | Arctic-Boreal and Boreal | Antarctic | Subantarctic | Bipolar-epiplanktonic | Cosmopolitan | Warm water | Deep sea | Total | |
---|---|---|---|---|---|---|---|---|---|---|
( ) = Bipolar subarctic and subantarctic. | ||||||||||
Coelenterata | ||||||||||
Trachy- and Narcomedusae | 4 | 10 | 3 | 6 | 90 | 21 | 134 | |||
Siphonophora: Calycophorae. | 2 | 1 | 64 | 5 | 72 | |||||
Physophorae. | 1? | 2 | 29 | 2 | 34 | |||||
Ctenophora. | 1 | 2 | 2 | 1 | 2 | 69? | 3 | 80? | ||
Nemertea | 52 | 52 | ||||||||
Polychaeta | ||||||||||
Tomopteridae | 1? | 4 | 1 | 38 | ? | 44 | ||||
Chaetognatha. | 2 | 2 | 2 | 18 | 6 | 30 | ||||
CRUSTACEA | ||||||||||
Cladocera. | 1 | (3) | 3 | 7 | ||||||
Copepoda. | 7 | 10 | 32 | 5 | 1(5) | 10 | 489 | 195 | 754 | |
Amphipoda | ||||||||||
Hyperiidea | 2 | 10 | 2 | 250 | 28 | 292 | ||||
Gammaridea. | 7 | 20 | 17 | 44 | ||||||
Euphausiacea | 6 | 2 | 7 | (1) | 46 | 23 | 85 | |||
Mollusca | ||||||||||
Pteropoda | ||||||||||
Thecosomata. | 2 | 1(1)? | 44 | 3 | 51 | |||||
Gymnosomata | 1 | 2 | (1)? | 37 | ? | 41 | ||||
Heteropoda. | 90 | 90 | ||||||||
Tunicata | ||||||||||
Appendicularia. | 2 | 1 | 6 | 1 | 48 | 3 | 61 | |||
Thaliacea | ||||||||||
Doliolidae | 1? | 11 | 12 | |||||||
Salpidae | 1 | 24 | 25 | |||||||
Pyrosomidae. | 8 | 8 | ||||||||
Total | 23 | 35 | 71 | 19 | 17 | 15 | 1378 | 358 | 1916 |
The greater number of species in the antarctic region over the arctic is also evidenced by littoral benthic life. But here the reason seems to be an historic one and not a matter of pelagic larvae or adults being transported thence by currents, for the Antarctic Continent is an isolated one and an extraordinarily large endemic population exists on its shores. Whatever the reason may be, it is of interest to note that, though warm or temperate waters appear favorable to species development, yet paradoxically the Antarctic possesses a littoral fauna richer in species than the Arctic shores of the Northern Hemisphere although the antarctic environment is colder than the arctic.
Oxygen
Oxygen is indispensable to the maintenance of the life processes of all organisms. It is available for normal metabolic activities of nearly all organisms only when it is in solution in a free state. A very few forms, notably the anaerobic bacteria, are able to carry on intermolecular respiration whereby the oxygen bound in the complex molecule of organic substance is made available as a source of energy. Therefore, biologically, free oxygen is comparable to carbon dioxide in being one of the two most important dissolved gases in the sea.
There are about 200 ml of oxygen in a liter of air as opposed to a maximum of about 9 ml in a liter of sea water. This is a great boon to air-breathing life, but with this advantage go also some disadvantages resulting from the need of maintaining moist respiratory surfaces in a desiccating environment. Aquatic and atmospheric respiration are similar in that the oxygen requires water as a respiratory medium; but in the former it should be noted that the oxygen-laden water is typically passed freely over the surface of relatively exposed respiratory organs or surfaces. Hence, although the concentration of oxygen reaching the respiratory surface is small, there is some compensation in its being rapidly replenished with complete flushing and aeration of the surfaces.
More oxygen can be dissolved in fresh water than in sea water, which should be advantageous to fresh-water animals; but, on the other hand,
The rate of metabolism is roughly doubled by a 10° increase in temperature. Therefore, during winter or in deep cold water, owing to the decreased rate of metabolism, much less food is required for repair following this slowing up of the katabolic processes. This reduced requirement must have an important bearing on the survival of many forms during winter when little food is being produced by the plants. The amount of oxygen used is somewhat different for different marine animals.
The order of magnitude of oxygen consumption in milliliters per gram of wet weight per hour within a temperature range of 17° to 25°C for various groups of marine invertebrates is as follows (averages from data of Fox, 1936, and compilations of Heilbrunn, 1937): Protozoa (Colpidium) 2.0; Coelenterates (jellyfish and ctenophores) 0.005; Echinoderms (various) 0.026; Annelids (various) 0.017; Crustacea (shrimps) 0.181.
For various marine bacteria the rate of oxygen consumption is very high compared to the above figures, being of the order of about 110 ml/g/hr at 22°C and depending upon nutrient conditions (ZoBell, 1940).
The great differences in the amounts of oxygen used per gram of wet weight are related to the great diversity in the water content of the living organisms. For example, low values found in coelenterates are correlated with the small percentage of solid organic material. Norris (in Hyman, 1940), for instance, found that the organic matter constituted only 0.85 per cent of the jellyfish Aequorea. The stage of development of some animals is also a factor in the rate of respiration. At 10°C Stage V of Calanus finmarchicus was shown to consume 0.25 ml of oxygen per thousand individuals per hour while adult females consumed 0.40 ml of oxygen per thousand individuals per hour (Marshall, Nicholls, and Orr, 1935). In recalculating the data of these investigators so as to present the oxygen consumption per hour in relation to weight in grams—using the average dry weight 27.35 mg/100 individuals of Stage V (Marshall, Nicholls, and Orr, 1935, p. 805), and considering the dry weight at 25 per cent of wet weight as Heilbrunn has done in his compilations—we arrive at an oxygen consumption of 0.228 ml/g/hr. If these figures present an accurate picture, we must assume that the metabolic rate of copepods is relatively high compared to other invertebrates, but this is in keeping with their very active lives. The figures arrived at for oxygen consumption by this species at Woods Hole are even considerably higher, namely 0.896 ml/g/hr at 15.5°C (see below). These values are higher than the ones found for certain marine fishes (Wells, 1935), the maximum oxygen consumption for Fundulus at 16°C being 0.220 ml/g/hr and for Girella at 20°C 0.242 ml/g/hr.
The concentration of oxygen in the sea is not only very much less per unit volume than in the air but there is also very much greater irregularity in its distribution and in some instances very sharp gradients may exist. The range of oxygen may be from 0 to 6.4 ml/l over a depth range of only 10 m, and in such rather isolated instances as in stagnant fjords the oxygen deficiencies are markedly reflected in the fauna.
The physical factors involved in the concentration, renewal, and distribution of oxygen in the sea are discussed elsewhere, and from these we note that on the whole the ocean, even in abyssal depths, is well supplied with oxygen for aquatic breathing organisms. We may therefore consider that oxygen is not, as a rule, a determining factor in the distribution and movements of most marine life. However, the irregularities in oxygen content and distribution referred to above are none the less highly important and, in many restricted instances, the low supply of oxygen and the hydrogen sulphide associated with this condition exclude all but anaerobic organisms on or near the bottom. Under these circumstances there may be not only an exclusion of animal life but a wholesale destruction of aerobic forms living at higher levels when temporary disturbances resulting from storms or surface cooling cause an upward displacement of these poorly aerated waters. This is witnessed periodically in certain Norwegian “threshold fjords” where a shallow sill prevents free water exchange with the sea and where summer heating and influx of fresh water have established a strong stratification of the water (pp. 147 and 802).
The Black Sea, with a maximum depth of 2104 m, being isolated from free circulation with the Mediterranean by the Bosporus Ridge, which extends upward to a depth of about 40 m, is illustrative of a condition where a more permanent biological climax has been reached. This high ridge precludes all opportunity of renewal of bottom water by oxygen-laden water from an outside source, and the accumulation of fresh water together with thermal conditions in the upper layers preclude any very deep aeration through convection currents. Decomposition of the rich organic material accumulating on the bottom from the productive surface layers has used up all of the free oxygen so that hydrogen sulphide extends upward from the bottom forming a layer of toxic water over 1800 m thick so that the lower limit of animal life is about 130 to 190 m (fig. 237). Only anaerobic bacteria can exist in the deeper portion of this environment, which constitutes about five times the volume of the upper portion capable of being inhabited by other organisms. These rigorous conditions, coupled with a reduced salinity, have led to an impoverished fauna with elimination of stenohaline and stenothermic species.
Very little is known regarding the relationship that exists between the oxygen-deficient or minimum layer (pp. 686–728) characteristic of
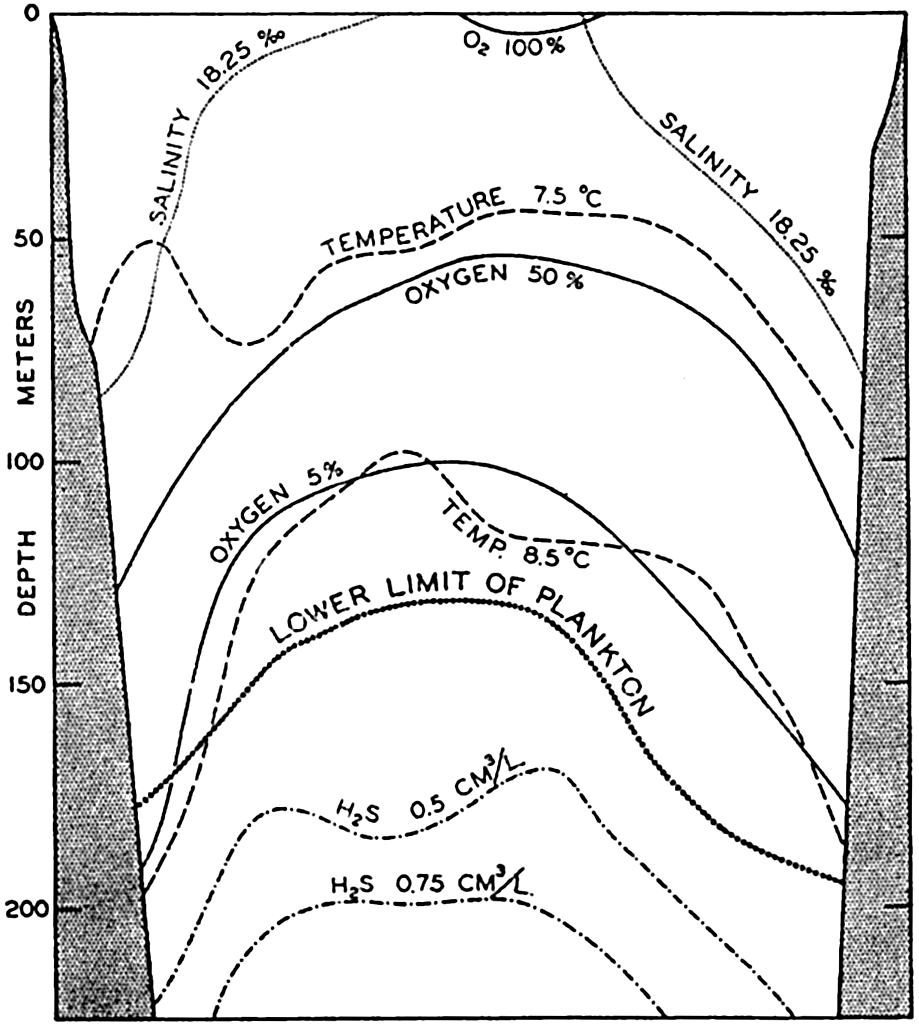
A section through the Black Sea to show hydrographic and biological conditions. (Modified from Nikitin.)
Schmidt (1925b) found that at a depth of 300 m in the Gulf of Panama, where there was only a 2 per cent oxygen saturation of water, plankton was about ten times more abundant than at the same depth in the Caribbean Sea with a saturation of 50 per cent. Oxygen determinations given in per cent may be misleading since they are a function of temperature and do not necessarily show the respiratory value associated with diminished metabolic rate in cold waters. The oxygen requirements of typically deep-sea plankton animals have apparently not been investigated.
Bibliography
“American Association for the Advancement of Science” . 1939. The migration and conservation of salmon.A.A.A.S. Pub.no. 8, F. R. Moulton, ed., 106 pp., 1939.
Barnes, T. C.1932. “Salt requirements and space orientation of the littoral isopod Ligia in Bermuda.” Biol. Bull., v. 63, p. 496–504, 1932.
Bigelow, Henry B.1926. “Plankton of the offshore waters of the Gulf of Maine.” U. S. Bur. Fisheries, v. 40, (for 1924), pt. 2, 509 pp., 1926.
Bigelow, H. B., and M. Sears. 1939. “Studies of the waters of the continental shelf, Cape Cod to Chesapeake Bay” . Museum Comp. Zool., Mem., v. 54, p. 189–378, 1939.
Blegvad, H.1929. “Mortality among animals of the littoral region in ice winters.” Danish Biol. Sta., Repts., v. 35, p. 51–62, 1929.
Bogorov, B. G.1932. “Materials on the biology of the copepods of Barents and the White Seas.” U.S.S.R., State Oceanogr. Institute, Bull.no. 4, English summary, p. 14–16, Moscow, 1932.
Brauer, A.1906. “Die Tiefsee-Fische.” Deutsche Tiefsee-Exped. Valdivia, Wiss. Erg., v. 15, Teil I, 432 pp., 1906.
Buch, K., and S. Gripenberg. 1932. “Über den Einfluss des Wasserdruckes auf pH und Kohlensäuregleichgewicht im grosseren Meerestiefen.” Conseil Perm. Internat. p. l’Explor. de la Mer, Jour. du Conseil, v. 7, p. 233–245, 1932.
Clarke, G. L.1933. “Diurnal migration of plankton in the Gulf of Maine and its correlation with changes in submarine irradiation.” Biol. Bull., v. 65, p. 402–436, 1933.
Clarke, G. L.1934. “Factors affecting vertical distribution of copepods.” Ecological Monographs, v. 4, p. 530–540, 1934.
Clarke, G. L.1936. “On the depth at which fish can see.” Ecology, v. 17, p. 452–456, 1936.
Clarke, G. L., and D. D. Bonnet. 1939. “The influence of temperature on the survival, growth, and respiration of Calanus finmarchicus.” Biol. Bull., v. 76, p. 371–383, 1939.
Coe, W. R.1938. “Primary sexual phases in the oviparous oyster (Ostrea virginica).” Biol. Bull., v. 74, p. 64–75, 1938.
Damas, D.1905. “Notes biologiques sur les copepodes de la mer Norvégienne.” Conseil Perm. Internat. p. l’Explor. de la Mer, Pub. de circonstance, no. 22, 23 pp., 1905.
Davidson, F. A., and Samuel Hutchinson. 1938. “The geographic distribution and environmental limitations of the Pacific salmon (Genus Oncorhynchus).” U. S. Bur. Fisheries, Bull., v. 48, p. 667–692, 1938.
Davis, W. M.1928. “The coral reef problem.” Amer. Geogr. Soc., Spec. Pub. 9, 596 pp., 1928. New York.
Doudoroff, Peter. 1942. “The resistance, reactions, and acclimatization of some marine fishes to changes of temperature.” Biol. Bull., v. 83.
Ekman, Sven. 1935. “Tiergeographie des Meeres.” Akad. Verlagsgesellsch. M. B. H., Leipzig, 542 pp., 1935.
Elsey, C. R.1934. “The Japanese oyster in Canadian Pacific waters.” Fifth Pacific Sci. Congr., Canada, 1933, Proc., v. 5, p. 4121–4127, 1934.
Elsey, C. R., and D. B. Quayle. 1939. “Distribution of oyster larvae from the 1936 spawning in Ladysmith Harbour.” Pacific Biol. Sta., Nanaimo, B. C., Progress Repts., no. 40, p. 8–10, 1939. Fisheries Research Bd., Canada.
Esterly, C. O.1917. The occurrence of a rhythm in the geotropism of two species of plankton copepods when certain recurring external conditions are absent.Univ. California Pub. in Zool., v. 16, p. 393–400, 1917.
Esterly, C. O.1919. Reactions of various plankton animals with reference to their diurnal migrations. Univ. California Pub. in Zool., v. 19, p. 1–83, 1919.
Farran, G. P.1911. “Resumé des observations sur le plankton. 2. Copepoda.” Conseil Perm. Internat. p. l'Explor. de la Mer, Bull. Trimestriel, p. 81–105, 1911.
Fish, C. J., and M. W. Johnson. 1937. “The biology of the zooplankton population in the Bay of Fundy and Gulf of Maine with special reference to production and distribution.” Biol. Board of Canada, Jour., v. 3, p. 189–322, 1937.
Fox, H. Munro. 1936. “The activity and metabolism of poikilothermal animals in different latitudes. 1.” Zool. Soc. London, Proc., p. 945–955, 1936.
Galtsoff, P. S.1930. “The role of chemical stimulation in the spawning reactions of Ostrea virginica and Ostrea gigas.” Nat. Acad. Sci., Proc., v. 16, p. 555–559, 1930.
Galtsoff, P. S.1938. “Physiology of reproduction of Ostrea virginica. 1. Spawning reactions of the female and male.” Biol. Bull., v. 74, p. 461–486, 1938.
Gardiner, A. C.1933. “Vertical distribution in Calanus finmarchicus.” Marine Biol. Assn. U. K., Jour., v. 18, p. 575–610, 1933.
Gardiner, J. Stanley. 1931. Coral reefs and atolls.London, Macmillan, 181 pp., 1931.
Gran, H. H.1902. “Das Plankton des Norwegischen Nordmeeres.” Rept. on Norwegian Fishery and Marine Investigation, v. 2, no. 5, 222 pp., 1902.
Grassi, B.1896. “The reproduction and metamorphosis of the common eel (Anguilla vulgaris).” Roy. Soc. London, Proc., v. 60, p. 260–271, 1896.
Grundfest, H.1932. “The sensibility of the sun-fish, Lepomis, to monochromatic radiation of low intensities” . Jour. Gen. Physiol., v. 15, p. 307–328, 1932.
Günther, A.1887. “Report on the deep-sea fishes collected by H.M.S. Challenger during the years 1873–1876.” Challenger Repts., v. 22, p. 1–335, 1887.
Hardy, A. C.1935. “The plankton of the South Georgia whaling grounds and adjacent waters 1926–1927, pt. 5., The plankton community, the whale fisheries, and the hypothesis of animal exclusion.” Discovery Repts., v. 11, p. 273–370, 1935.
Hardy, A. C., C. E. Lucas, G. T. D. Henderson, and J. H. Fraser. 1936. “The ecological relations between the herring and the plankton investigated with the plankton indicator.” Marine Biol. Assn. U. K., Jour., v. 21, p. 147–291, 1936.
Harvey, E. N.1920. “The nature of animal light.” Monographs on Exper. Biology.Philadelphia and London, Lippincott, 172 pp., 1920.
Harvey, E. N.1940. Living light.Princeton Univ. Press, 328 pp., 1940.
Heilbrunn, L. V.1937. An outline of general physiology.Philadelphia and London, W. B. Saunders Co., 603 pp., 1937.
Herdman, W. A.1923. The founders of oceanography.London, Edward Arnold & Co., 340 pp., 1923.
Hjort, J.1912. General biology. In Depths of the Ocean, by Murray and Hjort.London, Macmillan, 821 pp., 1912.
Hopkins, A. E.1937. “Experimental observations on spawning, larval development, and setting in the Olympia oyster, Ostrea lurida.” U. S. Bur. Fisheries, Bull., v. 48, p. 439–503, 1937.
Huntsman, A. G., and M. E. Reid. 1921. “The success of reproduction in Sagitta elegans in the Bay of Fundy and the Gulf of St. Lawrence.” Roy. Can. Inst., Trans., v. 13, pt. 2, p. 1–13 (Can. Biol. Bd., Studies, no. 5), 1921.
Hyman, Libbie H.1940. “Observations and experiments on the physiology of Medusae.” Biol. Bull., v. 79, p. 282–296, 1940.
Jespersen, P.1939. “Investigations on the copepod fauna in east Greenland waters.” Medd. om Grönland, Bd. 119, no. 9, p. 1–116, 1939.
Johnson, Martin W.1937. “The production and distribution of zooplankton in the surface waters of Bering Sea and Bering Strait. Rept.” Oceanogr. Cruise U. S. Coast Guard CutterChelan1934–37, Pt. II (B), p. 45–82, 1937.
Johnson, Martin W.1939a. “Pseudodiaptomus (Pseudodiaptallous) euryhalinus, a new subgenus and species of Copepoda, with preliminary notes on its ecology.” Amer. Micros. Soc., Trans., v. 58, p. 349–355, 1939.
Johnson, Martin W.1939b. “The correlation of water movements and dispersal of pelagic larval stages of certain littoral animals, especially the sand crab, Emerita.” Jour. Marine Research,v. 2, p. 236–245, 1939.
Johnson, W. H.1938. “The effect of light on the vertical movements of Acartia clausi (Giesbrecht).” Biol. Bull., v. 75, p. 106–118, 1938.
Johnstone, James. 1908. Conditions of life in the sea.Cambridge Univ. Press, 332 pp., 1908.
Keeble, F. W., and F. W. Gamble. 1904. “The colour physiology of the higher Crustacea.” Roy. Soc. London, Phil. Trans., (B)v. 196, p. 295–388, 1904.
Kikuchi, Kenzo. 1930. “Diurnal migrations of plankton Crustacea.” Quart. Rev. Biol., v. 5, p. 189–206, 1930.
Kofoid, Charles A.1930. Factors in the evolution of the pelagic Ciliata, the Tintinnoinea. p. 1–39 in Contributions to Marine Biology, Western Soc. Naturalists, Stanford Univ. Press, 1930.
Lohmann, H.1903.
“Neue Untersuchungen über den Reichtum des Meeres an Plankton und über die Brauchbarkeit der verschiedenen Fangmethoden.”
Komm. z. Wissensch. Untersuch. d. Deutschen Meere in Kiel und d. Biologischen
Loosanoff, V. L.1939. Spawning of Ostrea virginica at low temperatures. Science, v. 89, p. 177–178, 1939.
Macdonald, Roderick. 1927. “Food and habits of Meganyctiphanes norvegica.” Marine Biol. Assn. U. K., Jour., v. 14, p. 753–784, 1927.
Marshall, S. M., A. G. Nicholls, and A. P. Orr. 1935. “On the biology of Calanus finmarchicus. pt. 6. Oxygen consumption in relation to environmental conditions.” Marine Biol. Assn. U. K., Jour., v. 20, p. 1–28, 1935.
Murray, John. 1895. Summary of the scientific results, obtained at the sounding, dredging and trawling stations of H.M.S. Challenger, Repts., Summary, pt. 2, p. 797–1608, 1895.
Murray, John. 1913. The ocean. London, Williams and Norgate, 256 pp., 1913.
Murray, John, and J. Hjort. 1912. Depths of the ocean.London, Macmillan, 821 pp., 1912.
Natland, Manley L.1933. “The temperature and depth-distribution of some recent and fossil foraminifera in the southern California region.” Scripps Inst. Oceanogr., Bull., v. 3, p. 225–230, 1933.
Nicholls, A. G.1933. “On the biology of Calanus finmarchicus. III. Vertical distribution and diurnal migration in the Clyde Sea area.” Marine Biol. Assn. U. K., Jour., v. 19, p. 139–164, 1933.
Nikitin, W. N.1931. “Die untere Planktongrenze und deren Verteilung im Schwarzen Meer.” Internat. Rev. d. ges. Hydrobiol. u. Hydrogr., Bd. 25, p. 102–130, 1931.
Orton, J. H.1920. “Sea-temperature, breeding and distribution in marine animals.” Marine Biol. Assn. U. K., Jour., v. 12, p. 339–366, 1920.
Orton, J. H.1937. Oyster biology and oyster culture.London, Edward Arnold & Co., 211 pp., 1937.
Pearse, A. S.1936. The migrations of animals from sea to land.Duke Univ. Press, 176 pp., 1936.
Pierantoni, Umberto. 1918. Les microörganismes physiologiques et la luminescence des animaux. Scientia, v. 23, Suppl., p. 43–53, 1918.
Prytherch, H. F.1929. “Investigation of the physical conditions controlling spawning of oysters and the occurrence, distribution, and setting of oyster larvae in Milford Harbor, Conn.” U. S. Bur. Fisheries, Bull., v. 44, p. 429–503, 1929.
Redfield, A. C.1941. “The effect of the circulation of water on the distribution of the calanoid community in the Gulf of Maine.” Biol. Bull., v. 80, p. 86–110, 1941.
Redfield, A. C., and A. Beale. 1940. “Factors determining the distribution of populations of Chaetognaths in the Gulf of Maine.” Biol. Bull., v. 79, p. 459–487, 1940.
Regan, C. Tate. 1926. “The pediculate fishes of the suborder Ceratioidea. Danish Dana Expeds.” 1920–22, Oceanogr. Repts., no. 2, 45 pp., 1926.
Regan, C. Tate. 1932. “Deep-sea angler fishes (Ceratioidea).” Dana Rept.no. 2, 113 pp., 1932.
Runnström, S.1927. “Über die Thermopathie der Fortpflanzung und Entwicklung mariner Tiere in Beziehung zu ihrer geographischen Verbreitung.” In Bergens Mus. Aarb., Naturv. rekke, no. 2, 1927.
Russell, F. S.1927. “The vertical distribution of marine macroplankton. v. The distribution of animals caught in the ring-trawl in the daytime in the Plymouth area.” Marine Biol. Assn. U. K., Jour., v. 14, p. 557–608, 1927.
Russell, F. S.1928. “The vertical distribution of macroplankton. VII. Observations on the behavior of Calanus finmarchicus.” Marine Biol. Assn. U. K., Jour., v. 15, p. 429–454, 1928.
Russell, F. S.1935a. “On the value of certain plankton animals as indicators of water movements in the English Channel and North Sea.” Marine Biol. Assn. U. K., Jour., v. 20, p. 309–332, 1935.
Russell, F. S.1935b. “A review of some aspects of zooplankton research.” Conseil Perm. Internat. p. l’Explor. de la Mer, Rapp. et Proc.-Verb., v. 95, p. 3–30, 1935.
Russell, F. S.1939. “Hydrographical and biological conditions in the North Sea as indicated by plankton organisms.” Conseil Perm. Internat. p. l’Explor. de la Mer, Jour. du Conseil, v. 14, p. 170–192, 1939.
Russell, F. S., and C. M. Younge. 1928. The seas.London, F. Warne & Co., 379 pp., 1928.
San Francisco Bay Marine Piling Committee (C. L. Hill and C. A. Kofoid, eds.). 1927. Marine borers and their relation to marine construction on the Pacific coast. Final report of the committee cooperating with the National Research Council and the American Wood-Preservers' Association. San Francisco, 357 pp., 1927.
Schmidt, Johannes. 1909. “The distribution of pelagic fry and the spawning regions of the gadoids in the North Atlantic from Iceland to Spain, based chiefly on Danish investigations.” Conseil Perm. Internat. p. l’Explor. de la Mer, Rapp. et Proc.-Verb., v. 10, pt. 4, 229 pp., 1909.
Schmidt, Johannes. 1925a. “The breeding places of the eel. Smithsonian Inst.” , Ann. Rept. for 1924, p. 279–316, 1925.
Schmidt, Johannes. 1925b. “On the contents of oxygen in the ocean on both sides of Panama.” Science, v. 61, p. 592–593, 1925.
Sömme, J. D.1934. Animal plankton of the Norwegian coast waters and the open sea. I. Production of Calanus finmarchicus (Gunner) and Calanus hyperboreus (Kröyer) in the Lofoten area. Repts. on Norwegian Fishery and Marine Invest., v. 4, no. 9, 163 pp., 1934.
Steuer, A.1910. Planktonkunde.Leipzig and Berlin, B. G. Teubner, 723 pp., 1910.
Steuer, A.1933. Bericht über die Bearbeitung der Copepodengattung Pleuromamma Giesbr. 1898 der Deutschen Tiefsee-expedition Valdivia. Thalassia (Istit. Italo-Germanico di Biol. Marina, Rovigno d’Istria), v. 1, no. 2, 48 pp., 1933.
Sumner, Francis B.1911. “The adjustment of flatfishes to various backgrounds. A study of adaptive color change.” Jour. Exper. Zool., v. 10, p. 409–506, 1911.
Sumner, Francis B.1934. Studies of the mechanism of color changes in fishes. p. 62–80 in James Johnstone Memorial Volume, Lancashire Sea Fisheries, Liverpool, England, 348 pp., 1934.
Sumner, Francis B.1935. “Studies of protective color change. III. Experiments with fishes both as predators and prey.” Nat. Acad. Sci., Proc., v. 21, p. 345–353, 1935.
Sumner, Francis B.1939. “Quantitative effects of visual stimuli upon pigmentation.” Amer. Naturalist, v. 73, p. 219–234, 1939.
Sumner, Francis B.1940. “Quantitative changes in pigmentation, resulting from visual stimuli in fishes and amphibia.” Biol. Reviews, v. 15, p. 351–375, 1940.
Thompson, W. F.1936. “Conservation of the Pacific halibut, an international experiment.” Smithsonian Inst., Ann. Rept. for 1935, p. 361–382, 1936.
Thompson, W. F., and R. Van Cleve. 1936. “Life history of the Pacific halibut. 2. Distribution and early life history.” Intenat. Fish. Comm., Rept.no. 9, p. 11–184, 1936. Seattle.
Utterback, C. L.1936. “Spectral bands of submarine solar radiation in the North Pacific and adjacent inshore waters.” Conseil Perm. Internat. p. l’Explor. de la Mer, Rapp. et Proc.-Verb., v. 101, pt. 2, no. 4, 15 pp., 1936.
Vaughan, T. Wayland. 1919. “Corals and the formation of coral reefs.” Smithsonian Inst., Rept. for 1917, p. 189–276, 1919.
Walford, Lionel A.1938. “Effect of currents on distribution and survival of the eggs and larvae of the haddock (Melanogrammus aeglefinus) on Georges Bank.” U. S. Bur. Fisheries, Bull., v. 49, Bull. no. 29, 73 pp., 1938.
Wells, Nelson A.1935. “The influence of temperature upon the respiratory metabolism of the Pacific killifish, Fundulus parvipinnis.” Physiol. Zool., v. 8, p. 196–227, 1935.
Welsh, J. H., F. A. Chace, and R. F. Nunnemacher. 1937. “The diurnal migration of deep-water animals.” Biol. Bull., v. 73, p. 185–196, 1937.
With, Carl. 1915. “Copepoda. I. Calanoida amphascandria.” Danish Ingolf Exped., v. 3, no. 4, 260 pp., 1915. Copenhagen.
Worthington, E. B.1931. “Vertical movements of freshwater macroplankton.” Internat. Revue d. ges. Hydrobiol. u. Hydrogr., Bd. 25, p. 394–436, 1931.
ZoBell, Claude E.1940. “The effect of oxygen tension on the rate of oxidation of organic matter in sea water by bacteria.” Jour. Marine Research, v. 3, p. 211–223, 1940.