Chapter 3—
Chloroplasts—Structure and Development
3.1—
Introduction
Plastids are organelles which are bounded by double membranes and which occur, as far as is known, in all cells of eukaryotic green plants at some stage, usually becoming modified according to their function. In their undifferentiated form they may remain as proplastids, which are characteristic of epidermal and meristematic cells, for example. In the green parts of plants the proplastids normally develop into chloroplasts, which are the site of photosynthesis, while in starchstoring organs they form amyloplasts which produce the starch grains. However these two functions are not mutually exclusive as most chloroplasts will form starch under appropriate physiological conditions and the exposure of starch-storing organs to illumination results in the amyloplasts forming some thylakoids and chlorophyll. In certain plant parts, such as flowers, fruits and some leaves, the thylakoids of the chloroplasts become degraded, forming chromoplasts, which contain large amounts of carotenoids, the pigments responsible for 'autumn colouration' and the characteristic colours of certain flowers and fruits.
As the whole range of plastid structure, composition, genetics and development has been extensively covered in the monograph by Kirk and Tilney Bassett (1967) this chapter will confine itself to the consideration of chloroplasts, on which recent work has centred. The work of the author's laboratory has concentrated on a study of the structure and development of chloroplasts in Phaseolus vulgaris (bean) and Zea mays (maize) and thus many of the examples are drawn from work with these plants in order to make a consistent account. This approach must induce some lack of balance, which is acknowledged, but a properly balanced account of this topic would virtually require a volume of its own. References have been selected primarily for their clarity and not necessarily for their priority.
It has been postulated that the eukaryotic cell gained its autotrophic capacity by the capture of a prokaryotic organism at an early stage in the evolutionary process (Stanier, 1970), that the trapped prokaryote became the chloroplast, and that the nucleic acid of the resultant chloroplast still retains an important genetic function (see also chapter 9). Although the genetic evidence indicates that the plastid DNA is transmitted from generation to generation independently of the nuclear genes, Bell (1970) has published electron microscopic evidence that proplastids may arise anew each generation from the nucleus. However, it is hard to reconcile this latter proposal with the evidence that both nuclear and plastid DNA contribute to the plastid genotype (see chapter 9).
3.2—
Chloroplast Structure
3.2.1—
Chloroplast Dimensions and Number
Beans possess chloroplasts typical of the sun leaves of higher plants in that they are discoid or lens-shaped with a diameter of about 5µm and maximum thickness of about 1 µm as may be seen in the electron micrograph in Fig. 3.1B, which is a vertical section through the disc. On the other hand observations of chloroplasts in living cells by phase contrast microscopy suggest that the maximum thickness of the chloroplast may be rather less (S. G. Wildman, personal communication). Under optimum conditions for growth, Phaseolus vulgaris averages 45 chloroplasts per palisade mesophyll cell and 32 per spongy mesophyll cell, giving about 8.3 × 108 chloroplasts per leaf and about 2.3 × 107 chloroplasts per cm2 of leaf. This latter number is similar to that of mature spinach leaves (Possingham & Saurer, 1969) although the number of chloroplasts per spinach mesophyll cell is more than ten times greater than for bean.
3.2.2—
Chloroplast Fine-Structure
The following interpretation of chloroplast fine structure is based on transmission electron microscopy of thin sections of leaf material prefixed in 3% glutaraldehyde for 24 hours at 5°C, followed by fixation and staining with osmium tetroxide and post-staining with uranyl acetate and lead citrate. This technique is the most widely used, and, as glutaraldehyde is a relatively mild reagent, is considered to produce images most closely resembling the living plastid. Heslop-Harrison (1966) has written a critical account of the interpretation of chloroplast electron micrographs while the results of freeze-etch studies are considered in chapter 4.
The chloroplast envelope consists of two membranes (Figs. 3.1 and 3.2) the outer of which is unspecifically permeable to crystalloidal solutes. In contrast the inner membrane shows very specific permeability and has so far been shown to be the site of three specific anion translocation systems; (a) the phosphate translocator, facilitating a counter exchange of inorganic phosphate, 3-phosphoglycerate and dihydroxyacetone phosphate; (b) the dicarboxylate translocator, facilitating a counter exchange of dicarboxylic acids; and (c) the ATP translocator which is less active than the previous two and may be responsible for the entry of ATP in the dark (Heldt et al., 1972).
Within the inner membrane is a complex system of flattened sacs of membrane which were first termed thylakoids by Menke (1962). The thylakoids are closely associated in stacks (grana) which are shown in section for chloroplasts of bean and maize mesophyll in Fig. 3.1 (B and C). When seen from above the plane of the membrane the grana are essentially circular in outline with an average diameter of about 0.5 µm. Under optimum growing conditions bean grana
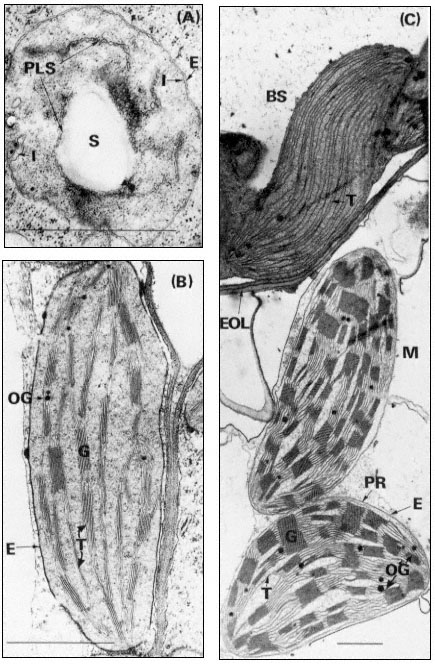
Figure 3.1
Plastids of bean and maize. A, proplastid from the primary leaf of a six day-old dark-grown
bean (× 42,000); B, chloroplast from the primary leaf of a bean grown for 14 days in the dark
and then transferred to continuous illumination (3 mW. cm–2 ) for 48 hours (× 29,000); C, bundle
sheath and mesophyll chloroplasts of maize grown under normal diurnal illumination (×12,500).
Scale lines represent 1 µm. Key to lettering: BS, bundle sheath; E, plastid envelope; EOL, electron
opaque layer; G, granum; I, invagination of envelope inner membrane; M, mesophyll; OG, osmiophilic
globule; PLS, porous lamellar sheet; PR, peripheral reticulum; S, starch grain; T, thylakoid.
contain up to 8 thylakoids while those of maize have up to 40. The thylakoids in each granum are continuous with those in other grana through intergranal thylakoids. In bean the granal thylakoids average 78%, and the intergranal thylakoids 22%, of the total thylakoid membrane (Bradbeer et al., 1974a). During chloroplast development the inner membrane of the envelope appears to give rise to the thylakoids by invagination (see Fig. 3.1A and p. 75) and it is possible that the space between the inner and outer membranes of the envelope is continuous with the whole of the thylakoid space of the chloroplast. Although the magnification of Figs 3.1B and C is such as to allow an overall impression of chloroplast structure, enough detail is visible to show that the intra-thylakoid space is electron-transparent, the thylakoid membrane is electron-opaque and the inter-thylakoid space seen in the grana between adjacent thylakoids is densely electron-opaque.
In the chloroplast the thylakoids are embedded, or suspended, in a matrix, the stroma, which has a somewhat granular appearance (Fig. 3.1). Within the stroma may be seen DNA fibrils and ribosomes (chapter 9), starch grains, osmiophilic globules and occasional extensive crystal-like structures. Such crystals can often be induced to appear in chloroplasts suspended in hypertonic media or in plants subjected to stress, although they have also been observed in plants grown under normal environmental conditions. It is thought likely that such crystals are composed of ribulosebisphosphate carboxylase (Larsson et al., 1973).
3.2.3—
The Mobile and Stationary Phases of Chloroplasts
From observations of chloroplasts in living cells by phase-contrast microscopy Wildman (1967) distinguished two components of the chloroplasts, a stationary phase which he equated to the thylakoid system and a mobile phase which he equated to the stroma. The mobile phase surrounds the grana and also penetrates the intergranal regions of the chloroplast. The mobile phase is always in some kind of motion though the intensity of the activity shows variability, even in the same chloroplast. In the reported investigations, observations were always made in cells which could be seen to be living by the presence of visible protoplasmic streaming. In such cells the mobile phase formed protuberances which sometimes broke away from the chloroplast and became indistinguishable from
mitochondria, and also mitochondria-like bodies appeared to fuse with the mobile phase. Subsequently Wildman and coworkers (1974) have reported that mitochondria-like bodies became stationary below tobacco chloroplasts in living cells and that starch grains subsequently appeared in similar positions in the chloroplasts. In the opinion of the present writer the interpretation that mitochondria give rise to starch grains cannot be substantiated. We have found that in plants grown under low light intensities each chloroplast has one or more mitochondria embedded in deep pockets close to the chloroplast margin but that no break in the chloroplast envelope occurs and there is always at least a thin layer of cytoplasm between chloroplast and mitochondrion (Montes & Bradbeer, 1976). It is possible that some of the observations of Wildman and colleagues may be explained by the development of the latter phenomenon.
3.2.4—
The Range of Chloroplast Structure
Quite early in the application of electron microscopy to plant structure the algae were found to show an interesting range of structural diversity of their chloroplasts. Subsequent studies on vascular plants established that a number of structural types were to be found in both cultivated and non-cultivated plants and furthermore that structural modification might be induced by mutation or by variation of the environmental conditions.
3.2.4.1—
The Algae
A monograph such as that of Dodge (1973) should be consulted for details of the range of chloroplast structure of this group. The characteristics of the chloroplasts have provided an important part of the basis for the taxonomic classification of the algae. In the green algae (Chlorophyceae and Prasinophyceae) the arrangement of the thylakoids tends to be basically similar to that in vascular plants and many of the differences may be associated with the non-discoid shape of the chloroplasts in most green algae. The simplest arrangement is found in the Rhodophyceae where the thylakoids occur as single sheets. In the Cryptophyceae the thylakoids tend to occur as pairs but the individual thylakoids in the pair do not appear to be fused to each other. The other algal classes have their thylakoids arranged in threes; in some cases (Dinophyceae, Euglenophyceae and Haptophyceae) the component thylakoids appear to be fused to each other while in other cases (Chrysophyceae and Phaeophyceae) the thylakoids do not appear to be fused. In two algal classes (Dinophyceae and Euglenophyceae) the chloroplast envelope consists of three membranes instead of two. In a number of classes (Bacillariophyceae, Chloromonadophyceae, Chrysophyceae, Cryptophyceae, Haptophyceae, Phaeophyceae and Xanthophyceae) the chloroplast is also surrounded by a sheath of endoplasmic reticulum which is usually continuous with the nuclear membrane. To complete this brief list of
the major peculiarities of algal chloroplasts it should be noted that the chloroplasts of many algae possess pyrenoids and eye spots.
3.2.4.2—
The Dimorphic Chloroplasts of C4 Plants
C4 plants form oxalacetate, malate and aspartate as the primary products of their photosynthetic CO2 -fixation in contrast to the more 'usual' C3 plants whose primary fixation product is 3-phosphoglycerate. Laetsch (1974) lists the following families of flowering plants as containing C4 species: Amaranthaceae, Aizoaceae, Chenopodiaceae, Compositae, Cyperaceae, Euphorbiaceae, Gramineae, Nyctaginaceae, Portulaceae and Zygophyllaceae. All of these families also contain C3 plants and there is the case of the genus Atriplex where the C4A. rosea will hybridize with the C3A. patula (Björkman et al., 1970). Apart from the difference in primary photosynthetic CO2 -fixation products C4 and C3 plants show other substantial differences in their biochemistry, physiology, anatomy and fine structure. Maize and bean will be discussed here as typical examples of C4 and C3 plants respectively
In the maize leaf the chloroplasts are concentrated in two concentric sheaths of cells around each vascular bundle. The inner sheath of cells is described as the bundle sheath and consists of equal numbers of large barrel-shaped cells and smaller cells which can be divided into two sorts on the basis of their dimensions (Montes & Bradbeer, 1975). Laetsch (1974) points out that the thick walls of bundle sheath cells adjoining mesophyll cells in C4 grasses possess an electronopaque layer (Fig. 3.1C). The bundle sheath chloroplasts possess abundant thylakoids which do not associate into grana, (Fig. 3.1C) and they normally contain starch grains. The plant from which the material was taken for Figure 3.1C had been stored in the dark for 24 hours prior to fixation to remove the starch so as to obtain a clear electron micrograph. In most cases, the bundle sheath chloroplasts of maize have been found to be completely agranal, although by modification of the environmental conditions the formation of grana can be induced (Bradbeer & Montes, 1976). In contrast Laetsch (1974) considers it normal for bundle sheath chloroplasts to show a small amount of thylakoid appression.
The outer sheath of cells is known as the mesophyll sheath and it contains chloroplasts similar to those of C3 plants in that they have grana. They are, however, different in that they do not normally contain starch grains. Not all C4 plants show such structural dimorphism of their chloroplasts. However all chloroplasts of C4 plants possess a system of tubules, the peripheral reticulum, (PR in Fig. 3.1C), which is associated with the inner membrane of the chloroplast envelope. There are reports of peripheral-reticulum-like membrane systems in chloroplasts of some cells of C3 plants (Laetsch, 1974). Circumstantial evidence assembled by the latter author suggests that the function of the peripheral membrane in C4 plants may be to facilitate transfer of metabolites between chloroplast and cytoplasm.
3.2.4.3—
The Effect of Environmental Conditions on Chloroplast Structure
The fact that plants grown in the complete absence of illumination form etioplasts (Fig. 3.2A and section 3.4.2) while those grown under diurnal illumination form chloroplasts establishes that light is an essential requirement for chloroplast development. When Björkman et al., (1972) compared plants of Atriplex patula which had been grown under three different irradiances: 20, 6.3 and 2 mW. cm–2 (in the waveband 400–700 nm), which are referred to as high, intermediate and low, they found that the high irradiance treatment yielded thicker leaves than the low, with more cells per leaf section and more chloroplasts per cell. The chloroplasts from plants grown under low irradiances were larger than those from high irradiance conditions and they contained more thylakoids and larger grana. The intermediate illumination gave intermediate results. The low irradiance-grown Atriplex plants were compared with plants adapted for growth on the floor of the Queensland rainforest where the daily quantum flux was about one-twentieth of that provided by the low irradiance treatment. The chloroplasts of these plants, Alocasia macrorrhiza, Cordyline rubra and Lomandra longifolia were found to be dark green, unusually large and irregular in outline and to contain very well developed grana of enormous size (Anderson et al., 1973). The grana were also irregularly arranged and apparently adapted for a maximum efficiency in light-trapping.
When maize plants were grown under very low irradiances (0.3 mW. cm–2 ), which nevertheless provided about six times the daily quantum flux received by the rainforest plants, some chlorophyll and photosynthetic CO2 -fixation developed even though the light compensation point was not exceeded and the leaves showed a net loss of CO2 followed by senescence and premature death (Bradbeer & Montes, 1976). Grana did not develop and both bundle sheath and mesophyll chloroplasts developed closely parallel arrangements of thylakoids which did not become appressed. Transfer of plants greened under very low irradiances to higher irradiances resulted in a partial recovery towards normal structure.
Research work in Belgium has shown that exposure of etiolated seedlings to electronic flashes of 1 millisecond in duration and given at 15 minute intervals brought about greening in which the resultant plastids were agranal with parallel unfused thylakoids. These flashed leaves showed the interesting property that, on transfer to continuous illumination, they initially did not show any photosynthetic oxygen evolution, but within 2 minutes of the commencement of illumination oxygen evolution was detected and a maximum rate was found after 6 minutes (Strasser & Sironval, 1972). When etiolated leaves are transferred to continuous illumination without any pretreatment with light the onset of oxygen evolution tends to be considerably delayed. An alternative treatment which has produced almost completely agranal plastids is exposure of dark-grown seedlings to continuous far-red irradiation at wavelengths longer than 700 nm (De Greef et al., 1971). For further discussion see page 80.
3.2.4.4—
Chloroplast Mutants
Many chloroplast mutants are known with defects in pigments or other components of structure or function. Some of the mutations are nuclear and show normal Mendelian inheritance whilst others show non-Mendelian inheritance and are considered to be mutations of the chloroplast DNA. Chloroplast mutations in algae can be maintained in heterotrophic culture and have been used for important research on chloroplast genetics, development and function (see e.g. Levine, 1969). In contrast, although there have been numerous investigations of individual chloroplast mutations in higher plants, the only substantial research collection of higher plant material with mutant chloroplasts is the barley mutant collection of D. von Wettstein's group in Copenhagen (von Wettstein et al., 1971). This collection has so far not been made generally available for studies on chloroplast development.
3.3—
Isolation of Chloroplasts
Although Hill in 1937, obtained chloroplast suspensions from Stellarsia media which were capable of O2 evolution under illumination, it was not until 1954 that Arnon et al., reported the occurrence of photosynthetic phosphorylation in isolated chloroplasts. These latter chloroplast preparations were capable of rates of photophosphorylation and electron transport similar to those assumed to occur in intact leaves, but they showed very low rates of CO2 fixation (<1 µmole CO2 fixed/hour/mg chlorophyll) compared with the rates found in intact leaves (200 µmole CO2 fixed/hour/mg chlorophyll). Little improvement in the rates of CO2 fixation by chloroplast preparations was obtained until Walker (1964) devised procedares which initially gave spinach chloroplasts able to fix 24.3 µmole CO2 /hour/mg chlorophyll. Subsequent modifications gave chloroplasts with improved rates of CO2 fixation and in the author's laboratory the following adaptation of Walker's method has been used routinely (Reeves & Hall, 1973). Washed spinach leaves are pre-illuminated for 30 minutes before use. 50 g of deribbed blades are rapidly cut up with a sharp knife to give pieces of about 2 cm–2 which are placed in a cooled perspex grinding vessel 6 cm × 5 cm × 25 cm. The leaves are then covered with 200 ml of fresh grinding medium which has been partially frozen to a slushy consistency. The grinding medium consists of 400 mM sorbitol, 10 mM NaCl, 5 mM MgCl2 , 1 mM McCl2 , 2 mM EDTA, 2 mM isoascorbic acid, 0.4% (w/v) bovine serum albumin and 50 mM 2-(N -morpholino) ethanesulphonic acid (MES) adjusted to pH 6.5 (at room temperature). The leaves are ground for 3 seconds with a Polytron PT20 with a PT35 head (Willems Kinematica GbmH, Lucerne, Switzerland) at a speed setting of 3.5. The resultant slurry is squeezed through two layers of butter muslin (cheese cloth) and the filtrate poured through eight more layers of muslin. The final filtrate is centrifuged in an MSE Super Minor bench centrifuge with a precooled
head. Rapid acceleration up to 4,000 × g, followed by braking by hand, (only to be attempted after training and with adequate safety precautions) enables the total centrifugation time to be less than 1 minute. The supernatant is discarded and the pellet gently resuspended with the aid of cotton wool and a glass rod in about 1 ml of solution identical to the grinding solution except that the isoascorbic acid is omitted and the MES is replaced by 50 mMN -2-hydroxyethylpiperzaine-N' -2-ethanesulphonic acid (HEPES) as buffer adjusted to pH 7.5 (at room temperature). The procedures are carried out at 0–4°C and the resultant chloroplast preparation is kept on ice. The total preparation time from cutting the leaves should be about 4 minutes. Most of the chloroplasts (60–80%) in such a preparation should be complete chloroplasts with a morphologically and functionally intact envelope and a high rate of light-dependent CO2 -fixation and O2 -evolution, similar to that found in vivo. They are considered to possess a full complement of unimpaired photosynthetic reactions although most of the reactions cannot be measured directly as the inner membrane of the envelope is impermeable to most of the intermediates which might be added to test these reactions. S. G. Wildman has demonstrated to me, by phase contrast microscopy, that most of the chloroplasts in this sort of preparation have lost their starch grains and therefore presumably possess resealed membranes.
The literature contains a multiplicity of published methods for obtaining a range of chloroplast preparations. To bring some order to the situation, Hall (1972) devised a scheme of nomenclature for these preparations, the main features of which are presented in Table 3.1. In this scheme the complete chloroplasts of Walker are classified as type A and the remaining types represent a succession of increased degradation. To the present writer the criteria for type B chloroplasts seem to be somewhat obscure and unsatisfactory and Walker (personal communication) is also sceptical about the validity of type B. Type B seems to have been proposed by Hall on the basis of older publications which had reported the preparation of apparently unbroken chloroplasts with impaired CO2 fixation. The other types of chloroplast preparation listed in Table 3.1 are more clearly defined and they correspond with the present state of knowledge.
For most chloroplast preparations investigators would seem to be best advised to prepare type A chloroplasts as the first step. Disadvantages of this procedure are that type A chloroplast preparations (a) contain a proportion of damaged and fragmented chloroplasts (normally 20–40% of the chloroplasts are not type A), (b) are contaminated with other organelles, (c) are contaminated with a substantial amount of cytosol, and (d) are obtained with a fairly low yield. In any further purification stages such as gradient centrifugation or washing there may be further damage to the chloroplasts and reduction of the yield. Consequently it is not yet possible to obtain a pure preparation of type A chloroplasts which is completely free from all other cellular components.
At the present time the application of similar techniques to those described above have yielded active preparations of type A chloroplasts from a very limited number of species (see e.g. Walker, 1971). For successful chloroplast
|
preparation it is usually necessary to modify the standard procedures, but despite modification some species have failed to yield appreciably active chloroplasts.
There are also two very different ways of obtaining plastid preparations which are worth noting. Wellburn and Wellburn (1971) devised a method of purifying structurally intact etioplasts by re-suspending the centrifugation pellet and passing this material through a loosely packed column of coarse Sephadex G-50. In vitro developmental studies have been carried out with such preparations (Wellburn & Wellburn, 1973), which show structually intact envelopes, although the biochemical properties of such etioplasts and the functional intactness of the envelopes do not appear to have been established so far. Alternatively, fractions rich in chloroplast material have been obtained by the non-aqueous homogenization and fractionation of freeze-dried leaf material (Stocking, 1971). Although important data have been obtained from non-aqueous preparations, the method is both technically difficult and hazardous, the preparations are impure and many of the biochemical reactions of the chloroplast are destroyed during the preparation.
3.4—
Chloroplast Development
Although the study of chloroplast development and the onset of photosynthesis in seedlings has attracted attention during essentially the whole of the twentieth century (Irving, 1910) it is in recent years that most interest has been shown. There have been two main methods of conducting this study, of which the first is essentially concerned with chloroplast development in plants grown under natural environmental conditions. Since natural conditions are normally neither constant nor consistent such studies have often been conducted in controlled environmental chambers set to a standard day length and standard conditions for day and night. When it became evident that illumination was the critical environmental factor controlling chloroplast development it became fashionable to study chloroplast development by allowing the seedlings to grow for an initial period of several days in complete darkness prior to their transfer to continuous illumination. Both approaches have their merits and it is clear to the present author that both are necessary to obtain an understanding of chloroplast development. Obviously seedling growth under natural environmental conditions shows the normal state of chloroplast development but it provides two main experimental difficulties. Firstly, under diurnal conditions there is a gradient of chloroplast development both within the plant and within the leaf, such that any experimental analysis is either very difficult or impossible. The second difficulty is that diurnal conditions make it difficult to distinguish the effects of illumination on chloroplast development. If seedlings are grown in continuous darkness for a sufficiently long period, their development reaches a stationary phase in which all of the developing plastids in a leaf or a section of a leaf show
the same stage of growth. On transfer to illumination the subsequent development then tends to occur in a synchronous manner, thus facilitating microscopic analysis and making biochemical analysis feasible. Such treatments may enable leaf chloroplast development to show synchrony like that obtainable in certain microbial cultures. It should also be pointed out that much progress in the study of photomorphogenesis has depended on illumination treatments of such darkgrown seedlings (Mohr, 1972). The behaviour of dark-grown seedlings in response to illumination does differ in a number of respects from seedlings grown under diurnal conditions of illumination, as discussed by Schiff (1975) for example.
3.4.1—
The Proplastid
Figure 3.1A shows an electron micrograph of a proplastid in a section of a primary leaf of a 6-day-old dark-grown bean seedling. The invaginations of the inner membrane of the envelope are interpreted as representing the formation of porous sheets of membrane which are shown in section. The proplastid also contains a starch grain and scattered ribosomes while the cytoplasmic ribosomes are more prominent and are apparently mostly present as polysomes.
3.4.2—
Etioplast Formation
Between 4 and 14 days of dark growth of Phaseolus vulgaris seedlings, the primary leaf primordia, which are already present in the dry seed, show a considerable amount of growth in increasing from a fresh weight of less than 1 mg to 30–40 mg while the cell number increases by more than 10 times and plastid number increases by 18 times (Bradbeer et al., 1974c). During this time the amount of membrane within the plastid increases considerably so that etioplasts like that in Fig. 3.2A are formed. The term etioplast was used first by Kirk and Tilney-Bassett (1967) and defines a structure which is typical of dark-grown seedlings, not normally found in plants grown under diurnal conditions of light and dark. In the bean etioplast about half of the membrane is organised in a regularly-arranged network of tubules called the prolamellar body with the remainder in concentrically arranged porous lamellar sheets (thylakoids). The prolamellar body shows a para-crystalline form and a knowledge of crystallography has contributed to the interpretation of its basic structure. In one plane the tubules form a mesh of hexagons, each individual hexagon being connected to the one immediately above by tubules arising from three alternate nodes, and to the one immediately below by tubules arising from the other three nodes (Weier & Brown, 1970). In planes cutting the hexagonal plane at 90º the arrangement of the tubules is approximately rectangular. Prolamellar bodies are frequently large and complex structures with evident discontinuities, but there are no published reports which account exactly for the structure of these large pro-
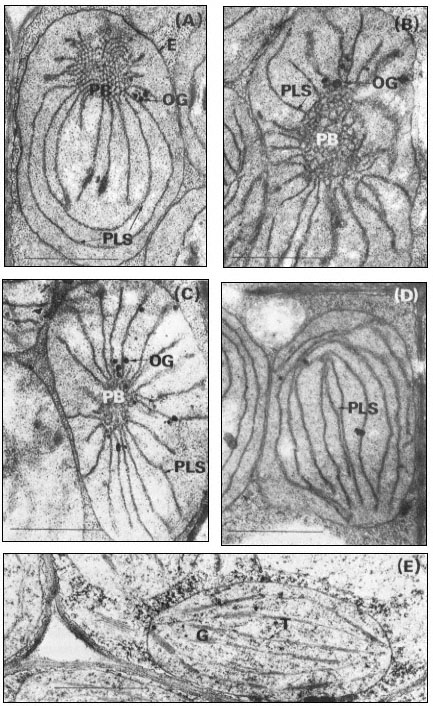
Figure 3.2
Stages of chloroplast development during the greening of the primary leaves
of 14-day-old dark-grown beans under continuous illumination of 3 mW. cm–2
A, no illumination; B, 105 minutes illumination; C, 4 hours illumination; D, 5
hours illumination; E, 15 hours illumination. Magnification × 25,000. Key
to lettering: PB, prolamellar body; other details as in Fig. 3.1.
lamellar bodies. Calculations based on measurements in the author's laboratory of electron micrographs of the prolamellar body structure described above show that 1 µm3 of the prolamellar body of 14-day-old dark-grown bean leaves should contain the equivalent of 44 µm2 of membrane.
From measurements of electron micrographs and the dimensions of the plastids determined by light microscopy it has been possible to follow the changes in the area of the lamellar sheets and of the volume of the prolamellar bodies during etioplast development. Determination of the number of plastids in the leaf then enables quantities per plastid (Bradbeer et al., 1974c) to be expressed on a per leaf basis as shown in Fig. 3.3. In Fig. 3.3 the area of membrane in the prolamellar bodies has been calculated on the basis stated above that 1 µm3 of prolamellar body contains 44 µm2 of membrane. As this factor is likely to vary during development according to the degree of contraction of the prolamellar body some inaccuracy is inevitable from this source for samples other than the 14-day-old one. In particular the slight apparent fall in the total membrane, shown in Fig. 3.3, after 14 days of dark growth may represent a contraction of the prolamellar body without any change in its membrane content. In the experiment shown in Fig. 3.3 membrane formation occurred between 6 and 14 days of growth. The porous lamellar sheets were formed first and the data are consistent with the presumed formation of the prolamellar bodies by some sort of condensation of these sheets (Weier & Brown, 1970). The area of the lamellar sheets reached a peak at 12 days after which the continued formation of the prolamellar bodies seems to have resulted in some consumption of the lamellar sheets.
During dark development of the etioplast most of the chemical components of the chloroplast are formed; for example all of the photosynthetic carbon cycle enzymes are present in the etioplast (Bradbeer et al., 1974c). Substances not yet detected in etioplasts which have received no illumination are chlorophyll, the chloroplast pigment-protein complexes and cytochrome b –559HP . The etioplasts of beans appear to reach the peak of their development by 14 days of dark growth while retaining an ability to form chloroplasts on illumination; however 17-day-old leaves with 75% of the etioplast membrane in the prolamellar body green only feebly and 21-day-old dark-green bean leaves fail to survive when transferred to illumination. It should also be pointed out that more rapid greening occurs in leaves younger than 14 days.
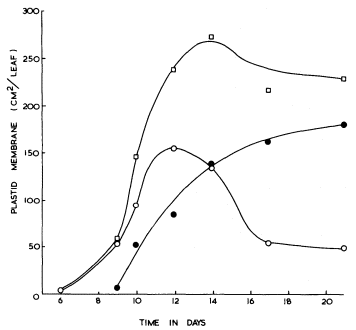
Figure 3.3
The formation of internal membrane during etioplast development in the
mesophyll of primary leaves of Phaseolus vulgaris during growth in
continuous darkness at 23°C


porous lamellar sheets;

3.4.3—
The Conversion of Etioplasts into Chloroplasts
The formation of chloroplasts in dark-grown leaves requires the provision of an appropriate amount and quality of illumination. Continuous illumination of 3 mW. cm–2 by fluorescent tubes in a growth cabinet was used for the electron microscopic study of 14-day-old dark grown beans shown in Fig. 3.2. For this material fixed with glutaraldehyde-osmium tetroxide, the paracrystalline appearance of the prolamellar body is retained for about 30 minutes after the beginning of illumination, and then between 30 and 60 minutes there is a change from a regular to an irregular appearance like that shown in Fig. 3.2B (Bradbeer et al., 1974a). Transformation of the prolamellar body appears to occur much more rapidly, usually within less than 1 minute of illumination, if the leaves are fixed with permanganate but this rapid change is commonly regarded as an artefact in structural terms. On the other hand, it may result from an early photochemical reaction in the etioplast which renders the paracrystaline nature of the prolamellar body less stable (Berry & Smith, 1971). Subsequently the volume of the reacted prolamellar body falls and the area of the thylakoid sheets increases as shown in Fig. 3.2C & D for 4 and 5 hours of illumination
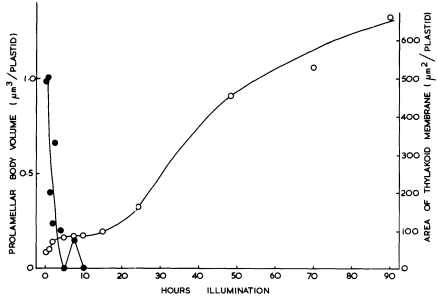
Figure 3.4
The effects of the transfer of 14-day-old dark-grown beans to continuous
illumination of 3 mW. cm–2 on the prolamellar body volume and the thylakoid area
of the plastids of the primary leaves.


respectively and in Fig. 3.4. Figure 3.4 shows that the fall in the prolamellar body volume and the increase in the area of the thylakoid sheets approximately correspond with each other with the actual increase of 21.5 µm2 thylakoid/plastid (43 µm2 membrane) accounting for the loss of 0.99 µm3 of prolamellar body per plastid (43.6 µm3 membrane). After 10 hours of illumination, appression of the thylakoids becomes more obvious, the formation of grana occurs, and the further increase in thylakoid membrane may be presumed to have resulted from de novo membrane synthesis. Figure 3.2E shows the stage of development after 20 hours of illumination and Fig. 3.1B that after 48 hours of illumination. During the course of bean chloroplast development under these conditions, de novo membrane synthesis results in an 8-fold increase in thylakoid area.
The illumination of 14-day-old dark-grown beans results in a synchronous development of the etioplasts in the primary leaf mesophyll cells. There is no division of these cells, less than 10% of the plastids divide, and the rather amoeboid etioplasts double in diameter to give typical discoid chloroplasts. The small amount of chloroplast division does not seem to be typical of developing leaves in which replication of chloroplasts is usual. Greening leaves show a lag before the onset of photosynthesis. The length of this lag depends upon the nature of the plant material, the conditions and duration of dark growth, the
conditions for greening, the intensity of the illumination used to assay photosynthesis and the sensitivity and nature of the method of assay. Consequently, published reports on the duration of this lag show a range of values; however, all agree that at least one hour of illumination, and usually more, is required before photosynthetic CO2 -fixation can be detected. For the bean leaves studied in the author's laboratory, the lag has been at least 5 hours, after which the photosynthesis of the developing chloroplasts becomes increasingly important as the source of the energy and the carbon requirements, of their own development (Bradbeer, 1976).
The effects of different light treatments on the form of the resultant chloroplast have been considered in an earlier section (3.2.4.3). There have been a number of studies in which light of different wavelengths has been used for the illumination of dark-grown seedlings in an attempt to determine an action spectrum of the light dependent reactions involved in chloroplast development. Henningsen (1967) obtained a sharp peak at 450 nm in the action spectrum for a stage termed vesicle dispersal in the development of bean plastids. Unfortunately this developmental stage may be another artefact of permanganate fixation as thylakoid extrusion does not involve such a stage when seen in glutaraldehydeosmic acid fixed material and studies with this latter fixative have not so far observed any corresponding action spectrum. However Henningsen's experiments have not been exactly replicated with the latter fixative and his data may well indicate a so far undefined photoresponse. Most studies of the effects of light quality on chloroplast development have used rather wide bands of wave-lengths and have concentrated on the role of phytochrome in chloroplast development. It has been shown in a number of laboratories that phytochrome has an important role in chloroplast development, and a number of aspects of chloroplast development are promoted by short treatments with red light and reversed by short exposure to far-red. In bean, the presumably-active form of phytochrome, Pfr (see chapter 12) promotes plastid expansion, plastid division, the formation of plastid membrane and the synthesis of chloroplast proteins (Bradbeer, 1971; Bradbeer et al., 1974b). These reactions may be regarded as 'slow' reactions in that they require several hours to become evident. In addition to phytochrome, 'slow' reactions may possibly also be effected by a red-light-absorbing photoreceptor other than phytochrome, and by a blue-light-absorbing photoreceptor. Most of the 'rapid' changes in etioplasts, which occur within three hours of illumination, do not seem to involve phytochrome; such changes as prolamellar body transformation and loss are sensitive to a wide range of wavelengths whilst thylakoid extrusion seems to depend on a red-absorbing photoreceptor other than phytochrome. Only two 'rapid' effects of phytochrome on etioplast fine structure have so far been defined; they are the Pfr-promoted crystallization of the prolamellar body in mustard cotyledons (Kasemir et al., 1975) and the Pfr-inhibited recondensation of the prolamellar body in bean primary leaves (Bradbeer & Montes, 1976). Although Wellburn and Wellburn (1973) have implicated Pfr in 'rapid' changes in isolated and
in situ etioplasts their method of analysis does not permit them to define these changes with any precision and their conclusions seem to require reconsideration.
3.4.4—
The Formation of Chloroplast Components in Greening Leaves
The transfer of dark grown plants to continuous illumination results in substantial increases in the amounts of most of the etioplast constituents which are also found in the chloroplasts (see for example Bradbeer et al., 1969; Gregory & Bradbeer, 1973). A few components, namely chlorophyll, the chloroplast pigment-protein complexes and cytochrome b– 559HP , which cannot be detected in dark-grown etioplasts, appear as a result of illumination.
The photosynthetic enzymes have almost exclusively been studied by determination of enzyme activity and thus any change in activity may result from either a change in the amount of enzyme protein or a change in the activity of the enzyme protein. Since, during the greening process, there is considerable protein synthesis it was considered that the simultaneous increases in enzyme activity probably resulted from protein synthesis. Subsequent investigations have established that enzyme activation is also responsible for a substantial part of the increase in the activities of certain enzymes in greening leaves.
Ribulosebisphosphate carboxylase is the most abundant protein in the chloroplast and probably consists of 8 large subunits (molecular weight about 5.2 × 104 ) and 8 small subunits (molecular weight about 1.3 × 104 ) which give a molecule of about 5.2 × 105 in molecular weight. By the use of two-dimensional polyacrylamide gel electrophoresis, Arron and Bradbeer (1975) found that the commencement of the synthesis of both subunits in bean leaves coincided with the beginning of illumination but that there was a lag before enzyme activity increased. Smith et al., (1974) reached a similar conclusion from experiments with barley in which they measured newly-synthesized ribulosebisphosphate carboxylase by labelling it during its biosynthesis and trapping the labelled substance with a specific antibody. In both bean and barley, synthesis of the enzyme occurs early in greening with a corresponding increase in enzyme activity, while late in greening after the enzyme synthesis has ceased considerable activation occurs. The mechanism of the activation is not known although the effect of illumination may be mediated by a small (molecular weight 5 × 103 ) constituent (Wildner et al., 1972).
In contrast, rather more is known about the activation of phosphoribulokinase and triosephosphate dehydrogenase, where a pretreatment of the extracted enzyme with 6 mM ATP prior to assay brings about activation, (Wara Aswapati et al., 1977). Activation may also be brought about by NADPH and sulphydryl reagents. On illumination of etiolated leaves, the activity of each of these enzymes shows a lag of several hours before it increases; however, preincubation of these extracts with ATP shows that the increases in activity seem to commence from the beginning of illumination (Fig. 3.5). It is concluded that synthesis of
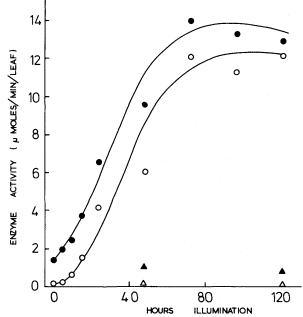
Figure 3.5
The development of phosphoribulokinase activity in primary
leaves of 14-day-old dark-grown beans on transfer to
continuous illumination of 3 mW.cm–2 .


after pretreatment of the extract with 6 mM ATP;


no pretreatment;




leaves left in dark. After Wara-Aswapati (1973).
these two enzymes commences with the beginning of illumination but that activity does not develop until photophosphorylation commences, thus accounting for the lag in Fig. 3.5 for the extracts which were not activated prior to assay. Thus, for the phosphoribulokinase in greening bean leaves Fig. 3.5 shows that there was a 10-fold increase in enzyme protein and a 9-fold increase in the enzyme activity of the protein, thus accounting for a 90-fold increase in total enzyme activity.
There is the further example of ATPase, which is a polypeptide complex in which illumination brings about an increase in enzyme activity with comparatively little new synthesis of the protein being apparent (Gregory & Bradbeer, 1975). In this case the effect of illumination seems to be more direct and is probably necessary for the onset of photophosphorylation rather than a result of it.
3.5—
Summary
The chloroplasts of higher plants are normally lens-shaped organelles with a diameter of about 5 µm and a maximum thickness of about 1 µm. They are
bounded by an envelope consisting of two membranes the outer of which is freely permeable to solutes while the inner shows very specific permeability. Within the inner membrane is a complex system of thylakoids (flattened sacs of membrane) which are closely associated in a number of stacks (grana), the thylakoid system itself being embedded in a matrix (stroma). Considerable variation in chloroplast structure is found in the algae. In plants which possess the C-4 pathway of photosynthetic CO2 fixation, dimorphism of chloroplasts is usual with agranal starch-containing chloroplasts occurring in the bundle sheath, which is a single layer of cells immediately surrounding the vascular bundles, while in the next outermost layer of cells (mesophyll sheath) are found most of the remaining chloroplasts, which possess grana and do not normally accumulate starch. Chloroplast structure may also be modified by environmental conditions or mutation.
Techniques for the isolation of chloroplasts for physiological and biochemical studies have been developed and the properties of the main types of chloroplast preparation are summarized in tabular form.
Proplastids occur in meristematic cells and are small with little membrane within the envelope. The direct conversion of proplastids to chloroplasts has been studied in plants grown under diurnal conditions of illumination although in most developmental studies seedlings have been grown in complete darkness at first so that the proplastids have developed into etioplasts. Etioplasts possess a substantial amount of internal membrane, some of which occurs as porous thylakoid sheets with the remainder condensed to give one or more regular lattices of tubules, the prolamellar bodies. On transfer of dark-grown seedlings to illumination the prolamellar body loses its regular structure and becomes converted into thylakoids. Subsequently de novo thylakoid synthesis occurs together with the formation of grana. Most of the chemical constituents of chloroplasts are already present in etioplasts. On illumination the missing components are synthesized, some enzymes are subjected to activation, most components undergo further de novo synthesis and photosynthetic activity develops after a lag. Some preliminary investigations have also been made of the effects of quality and intensity of irradiation on chloroplast development.
Further Reading
Anderson J.M., Goodchild D.J. & Boardman N.K. (1973) Composition of the photosystems and chloroplast structure in extreme shade plants. Biochim. Biophys. Acta325, 573–85.
Bradbeer J.W., Glydenholm A.O., Ireland H.M.M., Smith J.W., Rest J. & Edge H.J.W. (1974a) Plastid development in primary leaves of Phaseolus vulgaris. VIII. The effects of the transfer of dark-grown plants to continuous illumination. New Phyltol.73, 271–79.
Bradbeer J.W., Ireland H.M.M., Smith J.W., Rest J. & Edge H.J.W. (1974c) Plastid development in primary leaves of Phaseolus vulgaris. VII. Development during growth in continuous darkness. New Phytol.73, 263–70.
Heldt H.W., Sauer F. & Rapley L. (1972) Differentiation of the permeability properties of two membranes of the chloroplast envelop. In Proceedings of the 2nd International Congress on Photosynthesis Research, (eds G. Forti, M. Avron & A. Melandri) pp. 1345–55. Dr. W. Junk, The Hague.
Heslop-Harrison J. (1966) Structural features of the chloroplast. Sci. Prog., Oxf.54, 519–41.
Kirk J.T.O. & Tilney-Bassett R.A.E. (1967) The Plastids. W.H. Freeman & Co., London and San Francisco.
Laetsch W.M. (1974) The C4 syndrome: a structural analysis. Ann. Rev. Plant Physiol.25, 27–52.
Schiff J.A. (1975) The control of chloroplast differentiation in Euglena. In Proceedings of the 3rd International Congress on Photosynthesis Research, (ed. M. Avron) pp. 1691–1717. Elsevier, Amsterdam.