Chapter 7—
Geothermal Systems in Maturing Composite Cones
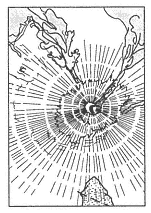
The most obvious volcanoes above sea level are the tall composite cones that occur in chains parallel to descending plate margins. Some of the more famous of these volcanoes are Mount Fuji, Mount St. Helens, Krakatau, and Vesuvius. Composite cones are large volcanoes that consist of multiple volcanic landforms such as interlayered pyroclastic rocks, lava flows, domes, and volcanic sediments. Composite cones are also known as stratovolcanoes or stratocones . Hundreds of composite cones overlie the Earth's subduction zones and may mark segmented arcs; they form island arcs and—on land where the crust is thicker—volcanic chains. Magmas are formed in the asthenosphere by the melting of descending plates as well as bits of the mantle and sediments overlying the plate at depths of 80 to 100 km; these magmas then rise buoyantly toward the Earth's surface.
Magmas reach the surface by fracture propagation and subsequent flow through dikes or by diapiric rise of blobs of viscous magma (Marsh, 1978), as is shown in Figs. 7.1 to 7.3. During the early history of a magmatic system, not many of these magma bodies will reach the surface to erupt or even to be emplaced at shallow depths. Magmas lose heat through conduction to adjacent crustal rocks; they become highly viscous and their rise toward the surface is halted. Either multiple periods of dike intrusion or the emplacement of diapiric magma bodies is required to create a zone or chain of heated pathways that enables succeeding magmas to reach the shallow crust (Fig. 7.1). Such a heating process allows larger, more viscous, slower moving magmas to aggregate and form bodies that reach the surface and erupt. However, shallower magma bodies associated with eruptions are ultimately the heat sources for geothermal systems.
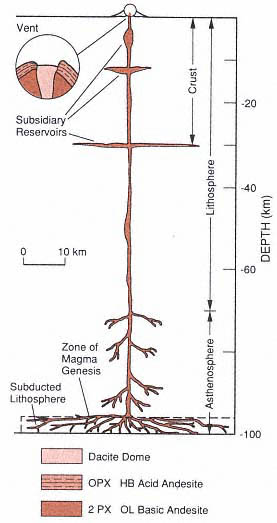
Fig. 7.1
Schematic cross-section for the system of
magma reservoirs below a medium-size composite
cone that is 8 km in diameter and has a volume
of 25 km3 . This cross-section is drawn to scale
except for the reservoir volumes, which are
exaggerated. The 70-km-thick lithosphere is
based on examples from the Aleutian Islands.
This model assumes 25% fusion followed by
80% extraction from a zone of magma genesis
with a volume of 250 km3 . OPX = orthopyroxene;
HB = hornblende; PX = pyroxene;
and OL = olivine.
(Adapted from Gill, 1981.)
Composite cones in volcanic arcs usually evolve with time. The volcanoes grow from individual simple cones into multiple cones, domes, and craters. Magmas become more silicic and explosive, and the volume of individual eruption sequences increases significantly. Networks of dikes, sills, and plutons are more pervasive and provide the structural framework that props up cones and shallow thermal sources. Surface manifestations above these intrusions become more evident, including fumaroles, the acid alteration of rocks in or near the summit crater, and hot springs located along the lower flanks of the cone. The recharge of meteoric water within a cone is important, not only because it contributes to the development of a hydrothermal system, but also because it can mask the thermal anomaly by an outward, rapid, near-surface movement of cold water.
This chapter discusses examples of composite cones and their activity and defines the surface indicators that can serve as guides to the geothermal systems within or below these volcanoes.
Distributions, Volumes, and Compositions
Distribution of Composite Cones in Volcanic Arcs
Volcanic arcs may break into segments, each of which is parallel to the arc trend and contains between two and a dozen volcanic centers (Stoiber and Carr, 1973; Marsh, 1979a; Carr et al ., 1982). Arc segments have been identified by mapping offsets in lines of volcanoes, contrasting volcano shapes and eruption styles, clusters of small basaltic volcanoes located behind the volcanic front near breaks, transverse fault zones, and clusters of large, shallow earthquakes at the segment boundaries (Carr et al ., 1982). Segmentation has been well-documented in Central America (Carr et al ., 1982), the Aleutians
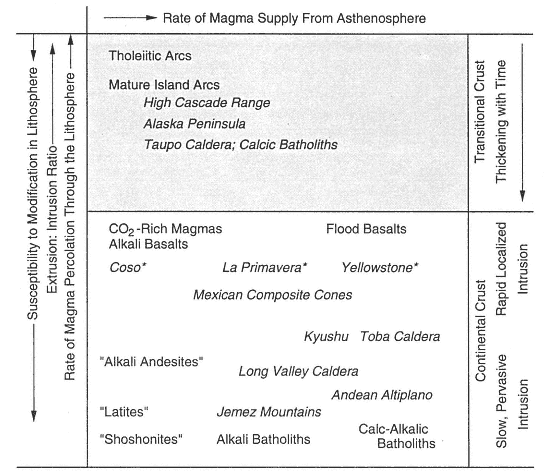
Fig. 7.2
Simplified two-dimensional diagram of a magma supply vs percolation rate model of lithospheric
magmatism. Axes are schematic and depict only the relative magnitudes of supply and the
modification of primitive magma in the systems shown. Asterisks indicate systems thought to be
characterized by the rapid transient injection of basalt at restricted crustal levels, which results in the
generation of rhyolite but little intermediate magma. In contrast, most continental and orogenic systems
may involve a diffuse injection throughout much of the lithosphere as well as subsequent mixing and
crustal mobilization, which in turn produce chiefly magmas of intermediate composition.
The degree of potential magma modification increases with increasing crustal thickness,
compositional contrast, and magma residence time.
(Adapted from Hildreth, 1981.)
(Marsh, 1979b), and the Mariana-Volcano Islands (Meijer, 1982).
Figure 7.4 shows a volcanic arc in Central America where zones interpreted as segments range in length from 55 to 260 km. Table 7.1 presents a comparison of segment length and volume for Central American volcanic arc segments. Stoiber and Carr (1973) reported that the volume of erupted lavas and pyroclastic rocks per kilometer increases with increasing segment length, from 1 km3 /km for the shortest (55 km) to 5.2 km3 /km for the longest (260 km). It has been proposed that arc segments reflect breaks and uneven surfaces within the descending plates (Carr et al ., 1982; Marsh, 1979a, b). Segment boundaries commonly
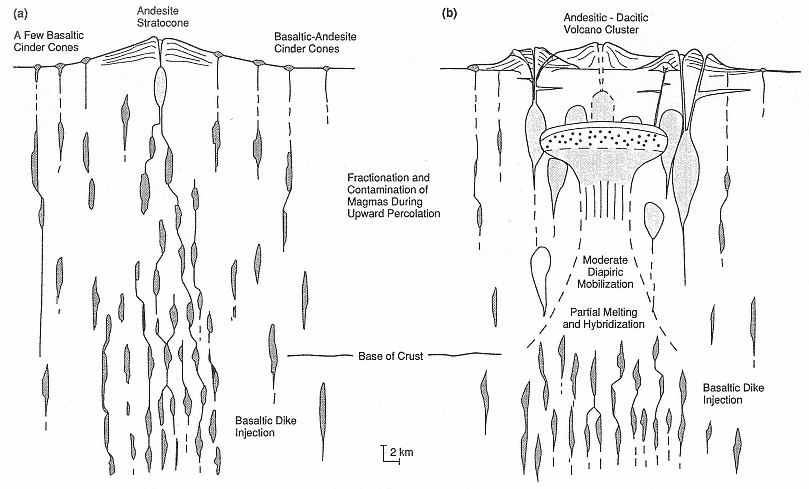
Fig. 7.3
Schematic cross-sections that depict two possible stages in the development of igneous systems in which tectonic
extension is subordinate and shallow. This model applies to (a) early and (b) intermediate stages of island arcs,
continental margin arcs, and mid-continental igneous systems.
Nearly all the heat for this system is supplied by basalt injection.
(Adapted from Hildreth, 1981.)
coincide with grabens that are oriented perpendicular to the plate boundary. Burkhart and Self (1985) interpreted these grabens as expressions of tectonic extension—rather than segmentation—of the plate.
The distance between composite cones located within adjacent arc segments is fairly uniform, and the volume of material erupted from each center is approximately proportional to that distance (Marsh, 1979a, b). For the Aleutian Island arc, this spacing is ~70 km (Marsh and Carmichael, 1974). Meijer (1982) noted that along the Mariana volcanic chain of the western Pacific, the spacing between volcanic centers ranges from 20 to ~80 km; this spacing is correlative with volcano size: the small volcanoes are the most closely spaced. Within segmented arcs, small, usually monogenetic cones may form at a distance of ~50 km behind and parallel to the volcanic front. These less voluminous cone clusters may develop 3 to 4 m.y. after the beginnings of arc volcanism (Marsh, 1979a).
Volcanic Eruption Rates and Relative Volumes for Magma Types in Composite Cones of Volcanic Arcs
Volcanic arcs and their composite cones contain a full spectrum of magma compositions—from basalt to rhyolite. Temporal variations in these compositions provide clues about the depth and size of the intrusive rocks that were their thermal sources. Compositional variations are affected by the rate of plate movement, the angle of plate descent, irregularities in the descending plate, crustal thickness, and the depth and residence time of the magma reservoirs.
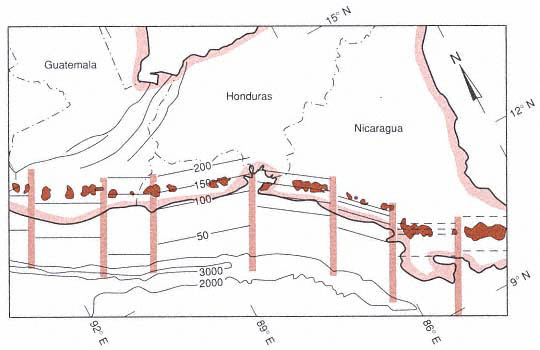
Fig. 7.4
The segmented volcanic front of Central America, in which active volcanic fields are shown as
shaded areas. Stippled vertical bars mark the transverse breaks in the arc. The thin, parallel lines
mark depths to the inclined seismic zones (contour interval is 50 km); offshore 1000-m
contours are depths below sea level.
(Adapted from Carr et al ., 1982.)
|
Another factor affecting the longevity of thermal sources is the extrusion rate for individual volcanoes and volcanic chains. This factor is difficult to evaluate because of the buried eruption sequences, erosion, and widespread distribution of pyroclastic products, but a number of studies have provided enough data to allow general estimates. Nakamura (1974) and Crisp (1984) reviewed data for volcanic output on a global scale and found that subduction-zone-related volcanoes produce from 0.4 to 0.75 × 106 km3 /m.y. Working with individual arcs, McBirney et al . (1974) and Sugimura and Uyeda (1973) concluded that the volume of material erupted for the Cascade Range and Japan, respectively, was ~5 km3 /m.y./km of arc. These general estimates were confirmed in a more detailed work by Sherrod and Smith (1990), who found that the extrusion rates in arc segments of the Quaternary Cascades volcanic arc range from 0.21 to 6 km3 /m.y./km of arc. Variations in the volume of material erupted from volcanoes of the Lesser Antilles and Central America may be related to both crustal thickness and rates of plate convergence; over the last 100,000 years, production rates have been 3.1 km3 /m.y./km of arc in Central America and 4 km3 /m.y./km of arc in the Lesser Antilles (Wadge, 1984). This relationship between convergence rate, crustal thickness, and magma types is shown in Table 7.2.
If an intermediate or silicic magma body—either small or large—is to rise buoyantly to crustal depths, it must be heated from below by basaltic magmas from the asthenosphere. Without this thermal boost, silicic magma chambers cool and solidify; they may never reach the upper crust (Lachenbruch et al ., 1976; Eichelberger, 1978). Fractionation and mixing of basaltic and silicic melts can produce the spectrum of magma types seen in composite cones. These compositional variations are controlled by the rate of magma supply, crustal thickness, rate of magma percolation through the crust, and extrusion to intrusion ratio (Figs. 7.2 and 7.3; Hildreth, 1981).
To calculate the number of shallow crustal magma bodies that might provide heat to geothermal systems, it is necessary to determine the relative volumes of magma types and their ages for each composite volcano and, if possible, for an entire arc. Central Cascade Range volcanism in North America began in the earliest Pleistocene with the eruption of widespread basaltic cones and flows and the construction of overlapping shield volcanoes (McBirney and White, 1982). Activity became more localized at centers from which more andesitic lavas and tephra were erupted. This activity formed the base upon which the large composite cones were constructed during the past million years. The volume measurements of
|
McBirney et al . (1974) indicated that most of the province consists of basaltic scoria cones and lava flows and that andesitic composite cones make up only 15% of the erupted material. Studies of Mount Jefferson indicate that the early basaltic activity produced >100 km3 , but that the cone-building stages involved only 25 km3 of andesitic magma (Fig. 7.5).
Clark (1983) found that the Three Sisters volcanic complex of the southern Cascade Range was erupted onto a broad base of basaltic lavas (57 km3 ), which included a much larger relative volume of andesite (30 km3 ) and rhyodacite-rhyolite (3.5 km3 ). Rhyodacitic domes erupted during the past 2300 years from aligned vents that cross the summit regions of the complex. These eruptions may have tapped only a small volume of a compositionally zoned, shallow magma chamber of much greater volume (Scott (1987).
By plotting volumes of erupted material vs SiO2 compositions for the Quaternary volcanic rocks of Japan, Aramaki and Ui (1982) demonstrated that changes along the arc may be related to both plate movement and crustal thickness. Arc segments that consist of composite cones, domes, and calderas are mostly andesite-dacite-rhyolite—all of which are indicative of shallow crustal magma bodies. Most of the rhyolitic materials are associated with large calderas. The basaltic segments are made up of pre-dominantly simple cones and lava flows (Fig. 7.6).
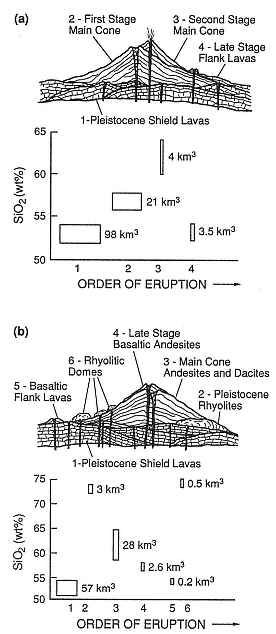
Fig. 7.5
Relative volumes of rock types associated with
Quaternary composite cones in the Cascade
Range of North America. (a) Mount Jefferson was
constructed during four main periods of activity;
rock volumes vs SiO2 content for each stage are
shown in the graph. (b) Three Sisters complex,
which is characterized by more siliceous rocks
than Mount Jefferson. Of the two, the Three
Sisters complex has more potential as a
geothermal resource.
(Adapted from McBirney and White, 1982.)
Inferred Intrusive Volumes and Their Depths below Composite Cones
Using a model originally developed for evaluating large-volume eruptions of silicic magmas and subsequent caldera collapse, Smith and Shaw (1975) determined that the volume ratio of magma chambers to erupted material is ~10:1. This conclusion was based on models of magma transport into the Earth's crust, exhumed intrusive-volcanic complexes, petrologic indicators, and geo-physical studies of active igneous systems. The authors later applied this model to the evaluation of all the high-grade geothermal systems in the U.S. Shaw (1985) took this model even further when he calculated volume-periodicity relations for explosive eruption activity in a variety of volcanoes—from composite cones located along plate boundaries to large mid-continental calderas. Most of the magma chambers below andesitic-dacitic cones examined in this study are located within the upper 4 km, and several are within 2 km of the crust.
Crisp (1984) and Wadge (1984) approximated intrusive-to-extrusive ratios for all volcano types, including composite cones and the associated domes and calderas along subduction zones (Table 7.3).
Eruption Phenomena and Deposits at Composite Cones
A composite cone consists of a stack of overlapping volcanic landforms deposited during a wide variety of eruptions that range from mild steam explosions to large Plinian eruptions accompanied by caldera collapse. The type of activity that produces any particular volcanic landform depends upon many factors, such as magma composition, volatile content, volume, and depth to underlying magma bodies, as well as the size and gravitational stability of the cone and its access to ground or surface water.
Composite cones usually evolve through time, as is displayed in Fig. 7.7; each successive eruption involves increased silica content in the magmas, shallower crustal magma bodies, and more energy. This process may last over several hundred thousand years but certainly less than a million years. Thus, many of the Earth's active composite cones are less than 200,000 years old. Mount Shasta in California, with a summit elevation of 3050 m, was constructed by four cone-building episodes during the last 250,000 years (Christiansen, 1985). The evolutionary model described in this section applies to most—but not all—composite cones; volcanoes are far too individual to allow absolute predictions of activity and products. For more detailed views of eruption processes, refer to Williams and McBirney (1979), Fisher and Schmincke (1984), and Heiken and Wohletz (1985).
Immature Stage
Lava Fountaining
Low-viscosity basaltic magmas erupt as lava fountains and basaltic lava flows. The fountains range from a few meters to >600 m in height (Fig. 7.8). They deposit welded spatter (bombs and ash) in either a circular or oval apron around a central vent or as ridges parallel to a fissure. The spray of low-viscosity (<103 -poise) basaltic liquid is driven by expansion of magmatic gases. Basaltic glass pyroclasts from lava fountains range from vesicular bombs a meter or more across to spheres of a few micrometers. The coarser pyroclasts are deposited within a few hundred meters of the vent; finer ash, including filamentous Pele's hair, is swept downwind and deposited as ashfall.
The structures associated with lava fountains are <100-m-high spatter ramparts and scoria cones composed of mixed welded spatter and basaltic ash. Pahoehoe and aa basaltic lava flows overflow from cinder cones and spatter ramparts or from fissures outside
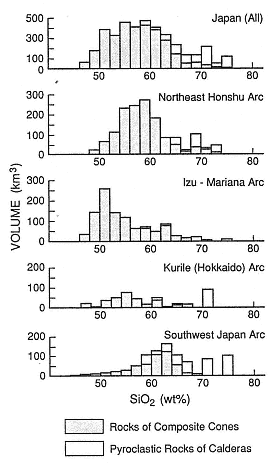
Fig. 7.6
Volume and weight percent of SiO2 from
Quaternary Japanese volcanic rocks. Patterned
portions represent lavas and pyroclastic rocks of
composite cones, lava domes, and pyroclastic
cones. Open portions indicate pyroclastic rocks
associated with large calderas.
(Adapted from Aramaki and Ui, 1982.)
|

Fig. 7.7
The evolution and geothermal potential of composite cones.
these structures, as can be seen at Kilauea (Fig. 7.9). These structures are often over-whelmed by lava flows, buried by small lava shields, or cut by pit craters. The volume of the lava flows in these eruptions greatly exceeds that of the pyroclastic rocks.
At this early stage of composite cone development, there are only simple, monogenetic cones composed of basaltic lavas. These may occur singly or in chains along prevolcanic fracture or fault systems. Of course, there are exceptions to this simple categorization; for example, Fedotov (1987) reported that 4750-m-high Kliuchevskoi Volcano in the Kurile-Kamchatka arc of the USSR is a basaltic cone with an annual magma output of 60 × 106 m3 .
Strombolian Eruptions
Explosive bursts of solidified and partly solidified bombs, blocks, and ash are termed Strombolian , from activity at Stromboli Volcano, which is located along the chain of volcanoes that make up Italy's Aeolian Islands. Well-documented Strombolian eruptions consist of "weak to violent ejection[s] of partly-fluid blobs" (MacDonald, 1972;
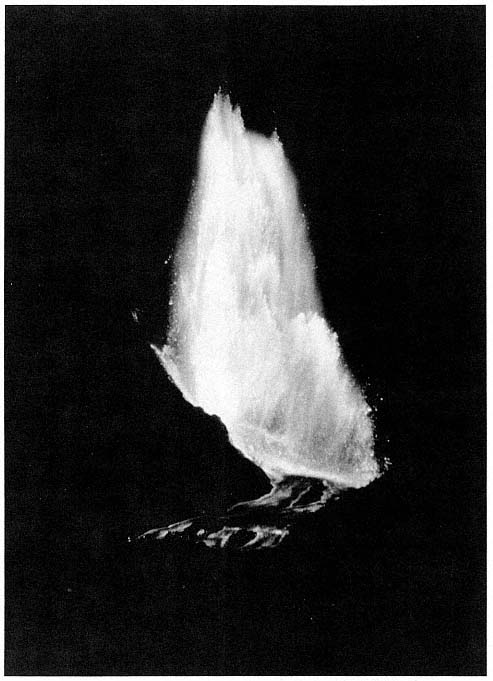
Fig. 7.8
This 300-m-high lava fountain occurred during the 1959–1960 eruption of Kilauea lki
in Hawaii. High flux, accompanied by a rapid release of magmatic gases, caused the
low-viscosity basaltic lava to fountain. This spray consisted of gases and droplets and
clots of lava. Similar lava fountains can occur during the early history of a composite cone.
(Photograph by the U.S. Geological Survey; Richter et al ., 1970.)
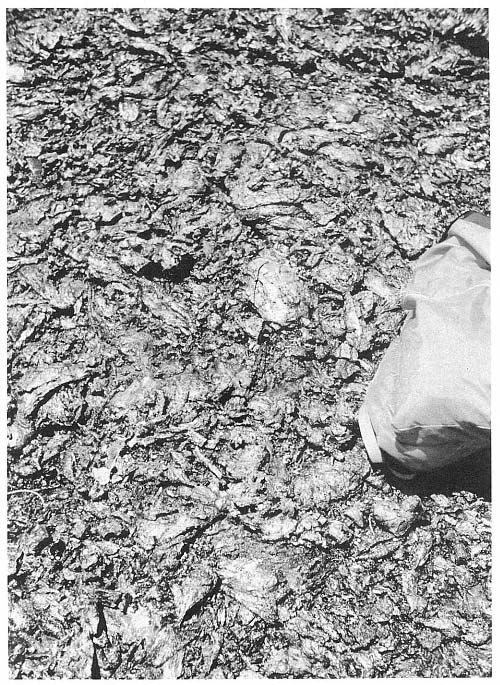
Fig. 7.9
Spatter rampart adjacent to a fissure vent at Kilauea Volcano in Hawaii. Partly molten bombs,
ranging from a few centimeters to several meters in diameter, fall out within a few tens or
hundreds of meters of the vent and form a resistant ridge or ring of welded scoria.
Self et al ., 1974; McGetchin et al ., 1974). Most of the pyroclasts fall ballistically around the vent and build up a scoria cone (Fig. 7.10); finer grained tephra is deposited on the cone and some is carried downwind. Fallout beds accumulate until they exceed their angle of repose, after which avalanches cascade down the flanks and into the crater (McGetchin et al ., 1974). Of the tephra erupted, ~50% is deposited in the cinder cone and 50% is deposited in fallout layers downwind from the cone (Heiken, 1978a).
Activity at scoria cones can rapidly alternate between lava fountaining, Strombolian bursts, and Vulcanian eruptions (discussed in the section on the submature stage of cone growth). Interbedded with the loose scoria fall and avalanche beds of many scoria cones are layers of welded scoria and finer grained phreatomagmatic tephra (for example, at Stromboli).
Pyroclasts in Strombolian deposits range from irregular, smooth-skinned, vesicular sideromelane droplets (basaltic glass) to blocky, crystalline, poorly vesicular tachylite pyroclasts. This spectrum of textural types is found in all size categories—from large bombs to scoria to fine ash.
Magma compositions represented in scoria cones vary from basaltic to basaltic andesite. Lava flows associated with Strombolian activity may be caused by overflows from crater lava lakes or eruptions from the cone flanks, which can even carry away part of the cone. Pahohoe, aa, and block lavas are all associated with scoria cones.
Submature Stage
This stage of growth includes the development of individual vents along lineaments. Magmas are mostly of intermediate compositions that comprise basaltic andesites,
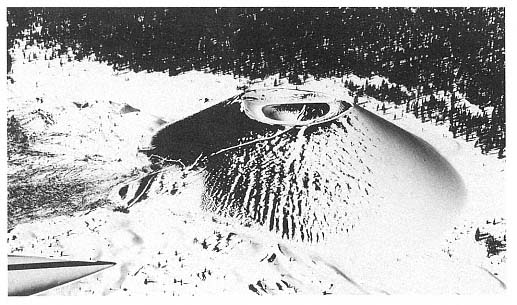
Fig. 7.10
Cinder Cone at Lassen National Park in California. This scoria cone is typical of cones that are
peripheral to the more silicic volcanoes of the Lassen area. Built during several periods of
eruptive activity during the last 400 years, this cone was last active in 1851 A.D. Cinder Cone
consists of outwardly dipping layers of several types of scoriaceous bombs and ash
that were deposited ballistically and by slumping of oversteepened slopes.
andesitic basalts, and andesites. As the volcano grows in elevation, it may affect local weather patterns; heavier precipitation occurring near the summit may saturate rocks or form snowfields and glaciers. With the presence of increased surface and groundwater on the volcano, the potential for hydrothermal activity and phreatic eruptions will also increase. The factors responsible for the trend toward Vulcanian activity are increasingly viscous magma and increasing amounts of groundwater.
Vulcanian Eruptions
A Vulcanian eruption is characterized by the moderate to violent ejection of solid or very viscous lava fragments in short, cannon-like bursts. Ash, fine ash, and gases are emitted and ascend to form a cauliflower-like eruption cloud. The eruption mechanism is not clear, but it appears to consist of alternating magmatic and phreatomagmatic processes. Occasionally, eruptions will shift back and forth between Vulcanian and Strombolian activity. They can produce eruption clouds that rise several kilometers above the vent as well as small pyroclastic flows and surges that flow down the cone flanks. These pyroclastic flow deposits may grade into laharic breccias on the lower flanks of the volcano—possibly as a result of condensation of the steam-particulate mixture. Vulcanian eruptions form scoria cones, craters in composite cones, and lava domes.
Vulcanian deposits consist of large blocks and bombs in a matrix of juvenile and non-juvenile ash. Heiken and Wohletz (1985) determined that the juvenile components may be vesicular (mostly magmatic) or blocky and nonvesicular (mostly phreatomagmatic). Nonjuvenile components are interpreted as fragments of a lava plug in the conduit, bits of dome lava, or fragments from the conduit and crater walls.
Nairn and Self (1978) reported that at Ngauruhoe volcano in New Zealand, cannon-like explosions and subsequent pyroclastic falls and flows resulted when a lava plug was pulverized in the conduit by a combination of magma degassing and vaporization of groundwater.
Volcanic Mudflows (Lahars)
A volcanic mudflow is a mixture of juvenile and nonjuvenile pyroclasts, lithic clasts picked up on the volcano surface, and a silt or mud matrix. Lithic blocks can range from pebble size to slabs that are tens of meters in diameter. Within the fine-grained matrix may be vesicles formed by trapped air or steam. Fisher and Schmincke (1984) and Crandell (1971) provide excellent detailed descriptions of volcanic mudflows.
Volcanic debris flows are nearly always associated with composite cones in submature or mature stages of growth. These flows can be initiated in several ways: (1) condensation of water vapor in cooling pyroclastic flows or surges (Wohletz, 1986), (2) phreatic eruptions of muddy tephra, (3) expulsion of water from crater lakes, and/or (4) snow and ice melt following emplacement of small pyroclastic flows. Lahar is a commonly used Indonesian term for a coarse, poorly sorted volcanic debris flow (van Bemmelen, 1949). Lahar deposits may coat the flanks of a cone, but they are generally confined to the canyons and valleys of the watershed. They may travel for distances of > 100 km if the volume is great enough and the stream gradient steep enough. Where there is a change of gradient—for example at the head of a plain below the volcano—lahars spread out to form fan-like sheets. Because they are generally confined to drainages, volcanic mudflows are interbedded with reworked pyroclastic deposits and eroded lava flows. Surfaces of debris flow breccias of any type can be smooth, but they can also be hummocky when they contain >1-m diameter blocks that have been carried along with the flow, as shown in Fig. 7.11. In most field situations it is difficult to distinguish between laharic deposits and debris avalanche deposits from volcanoes.
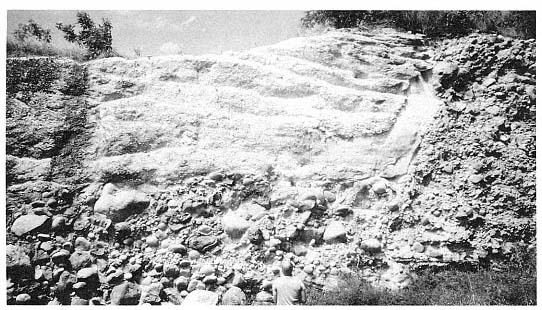
Fig. 7.11
A laharic breccia and a small, valley-filling ignimbrite exposed along the east coast of the
island of St. Lucia in the West Indies. Most of the outcrop consists of a matrix-supported
boulder-rich breccia deposited by a volcanic mudflow (lahar). The steep-walled, flat-floored
ravine cut into the breccia has been filled by a massive ignimbrite (the Qualibou Tuff).
Laharic breccias are massive and consist of boulders in a fine-grained, muddy matrix. Most are reversely graded, but some are normally graded; this feature depends on the bulk density of the fluid as well as the velocity and strength of the mudflow. All such breccias are very poorly sorted and thick-bedded deposits. Trees, shrubs, and grass, frequently ripped up by the mudflows in the upper reaches of a drainage, are carried along with the flow. The plants may or may not be charred, depending upon the volume of hot juvenile pyroclasts in the deposit; if the plants are charred, it might be possible to use carbon-dating techniques on the mudflow.
Mature Stage
At this stage of composite cone development, several new factors are evident.
· Magmas are more silicic, more volatile-rich, and thus more explosive.
· Magma bodies are more viscous, are larger, and are emplaced at shallow depths.
· Shallow hydrothermal systems have developed and there is acid alteration of the cone's core.
· The emplacement of shallow magma bodies and subsequent surface deformation, the great height of the cone, and hydrothermal alteration of interior portions of the cone cause instabilities that may lead to sector collapse. (a sector is a cone section that may fail structurally from summit to base.)
In the mature stages of composite cone development, the balance of construction and destruction is similar to the processes
exhibited during the eruption of Mount St. Helens in Washington: sector collapse, explosive eruptions, and subsequent slow dome growth, which may eventually fill the crater formed by collapse.
Plinian and Peléean Eruptions
Plinian and Peléean eruptions are associated with volatile-rich rhyolitic or dacitic magmas. The eruptions range in magnitude from pumice falls of <0.1 km3 to tens of cubic kilometers. These eruptions produce not only widespread pumice and ash falls but also extensive pyroclastic flows. Most of these eruptions are considered magmatic and are driven by the release of magmatic gases; however, many are also phreatomagmatic. (The best examples of the latter are associated with calderas in Italy and New Zealand.)
Plinian pumice fall and ashfall deposits systematically decrease in thickness with distance from the vent. Elliptical fallout patterns are possible unless there is no wind during the eruption—in which case the pattern is circular. Fallout plane-parallel beds drape the topography and are normally or reversely graded, depending upon the eruption energy, the winds, or both. Figure 7.12 shows a pumice fallout deposit at Tecuamburro Volcano in Guatemala. For a detailed description of all types of ashfall deposits, see Fisher and Schmincke (1984).
Fallout tephra is moderately to well sorted; its median grain size decreases downwind from the vent. Such a deposit is mostly composed of angular, vesicular pumice pyroclasts. The volume of vesicles and phenocrysts and the vesicles' shapes vary greatly between eruptions and even during a single eruption. A characterization of the vesicles and an analysis of phenocrysts can be used to interpret details of magma movement and eruption phenomena (Fisher and Schmincke, 1984; Heiken and Wohletz, 1985).
Pyroclastic flow and surge deposits from Plinian eruptions can be either small-volume deposits—limited mostly to drainages on the volcano slopes—or large volumes—tens of cubic kilometers or more—that form aprons radial to calderas. (Chapter 2 provides detailed descriptions of facies within pyroclastic flow deposits.)
Silicic Lava Domes and Flows
Rhyolitic, rhyodacitic, and dacitic lavas are erupted slowly and form bulbous, steep-sided domes and lava flows close to the vent area (Fink, 1987). Rose (1987) reports that Santiaguito in Guatemala has been erupting dacitic lava flows continuously for >60 years. The flow surfaces are marked by highly fractured, contorted lobes; however, within the flows, the lavas are flow banded, pumiceous and nonpumiceous, and glassy to crystalline (Fink and Manley, 1987). These domes are associated with craters but can also erupt from fissures.
Silicic domes make up an integral part of many composite cones, especially during the mature stages of cone growth. At the Three Sisters volcano in Oregon, for instance, silicic domes and flows and associated pyroclastic rocks erupted from 20 vents and have formed a 10-km-long chain across the summit of South Sister (Scott, 1987).
Domes within the summit craters of composite cones may be purely surficial, spreading out laterally from a rhyolite dike, or may be part of a plug that fills a large conduit to a depth of a kilometer or more. Volcán Santa Maria in Guatemala was cut in half during an explosive eruption in 1902. Twenty years later, a dome began to grow in the crater and has erupted 1 km3 of dacitic lava in 22 distinct flow lobes to date (Rose, 1987). This activity has not restored the ~5 km3 of material lost in the 1902 eruption, but the volcano continues to grow and maintains the cycle of destruction and construction characteristic of composite cones (Fig. 7.13).
The process of destruction was clearly demonstrated by collapse of the northern flank of Mount St. Helens during the eruption
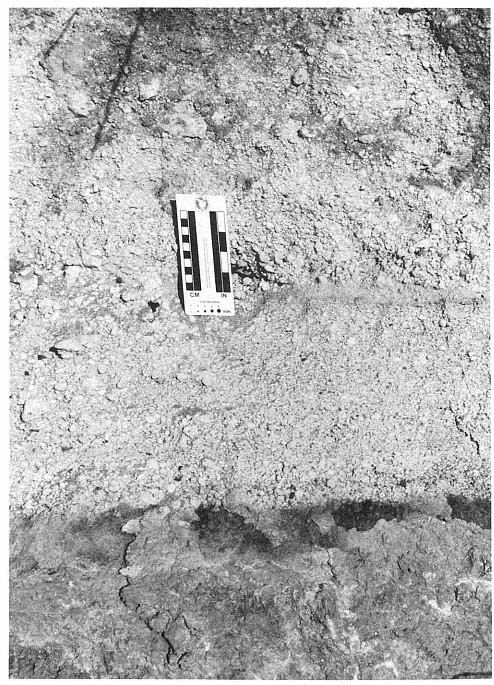
Fig. 7.12
This pumice fallout deposit is overlain by a massive ignimbrite from the same eruption.
An unnamed pyroxene pumice deposit in the Tecuamburro Volcano area of southeastern
Guatemala at this location consists of both a 10-cm-thick pumice fallout deposit, which
drapes underlying topography, and massive ignimbrites, which are thick within valleys
and canyons and thin or absent on ridges and hills.
of 1980. The collapse removed 2.3 km3 of rock as a rockslide and avalanche from the summit and core and left a large open amphitheater-like crater (Voight et al ., 1981). This space is now partly filled by a dacitic dome that continues to grow. This process is slowed only by intermittent periods of destruction or partial destruction by magmatic and phreatic explosions. In the three-year period after the crater was formed, 0.04 km3 of dacitic lava was erupted. Swanson et al. (1987) estimated that if the new magma is erupted slowly and continuously, ~0.01 km3 of dacite will be added each year to the dome (Fig. 7.14).
Kienle and Forbes (1976) noted that during the initial 1976 explosive activity at Augustine Volcano in Alaska, ash falls and pyroclastic flows swept the slopes, removing ~0.1 km3 of dome material. The cylindrical crater was refilled within a few hours by fresh dacitic lava.
Fumarolic Activity and Acid Alteration
Mature composite cones with summits high above the surrounding terrain are the sites for increased precipitation and infiltration of meteoric waters that feed vapor-dominated hydrothermal systems (Healy, 1976). Usually, fumaroles located near the summit form zones of acid alteration in the crater or along faults that cut the crater. Hot springs commonly issue from fractures, faults, or permeable beds near the base of the volcano. This type of hydrothermal system implies the presence of shallow young magma bodies within or below the base of the composite cone.
Explosive Phreatic Activity
If a hydrothermal system is perturbed by the injection of new magma at depth, the result may be explosive steam eruptions without the eruption of juvenile tephra. This type of activity is possible at many types of volcanoes and even in geothermal areas where there is no volcanic activity; however, it is
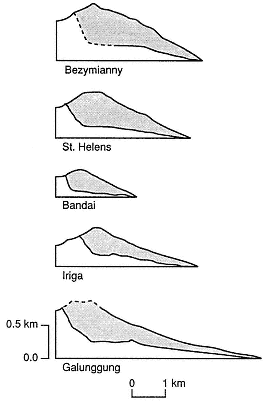
Fig. 7.13
Cross-sections of composite cones that
contain sector collapse craters.
(Adapted from Siebert, 1984).
most common in mature composite cones. Phreatic eruptions may occur without subsequent activity (such as at Soufrière de Guadeloupe in 1976–1977), or they may be precursors of a significant magmatic eruption (as at Mount St. Helens in 1980). Phreatic eruptions consist of intermittent or continuous explosive steam bursts and can form large craters.
The phreatic ashfalls observed at Soufrière de Guadeloupe are thin, fine-grained deposits with large blocks near the vent as well as small volcanic mudflow deposits composed of hydrothermally altered or weathered lithic clasts and mud (Wohletz and Crowe, 1978; Heiken and Wohletz, 1985). Intermittent explosive phreatic
activity is an important indicator of geothermal potential and is described in greater detail in Chapters 2 and 3.
Models of Composite Cones
To find and develop a hydrothermal system associated with a composite cone, it is necessary to understand the cone's structural framework, intrusive "plumbing," and thermal state. This information must be inferred from clues at the surface such as surface manifestations of hydrothermal activity, the age and composition of the volcano, and its fractures, faults, and hydrology (Sibbett, 1988). The ability to relate surface features to interior structure and thermal state depends on models that have been developed from geophysical models or through the examination of eroded composite cones. A variety of these models are discussed here; each is based on a different approach or tectonic setting.
Models Based on Mapping and Mining of Porphyry Copper Deposits in Deeply Eroded Composite Cones
Branch (1976) studied many composite cones in Papua New Guinea to determine if they contained volcanogenic ore bodies. Branch published a model of composite cones (shown in Fig. 7.15) that was based on his field observations of basaltic to andesitic volcanoes in various evolutionary stages. The composite cones developed over an island arc subduction zone in a region with a relatively thin crust. They were composed of interbedded lava flows, laharic breccias, and other pyroclastic deposits. Eruptive cycles were as short as 1 year or as long as 10,000 years; during the latest growth stages, the cones were permeated with shallow magma chambers, sills, and dikes. Hydrothermal systems developed during later stages extend into the core of the cone and through underlying basement
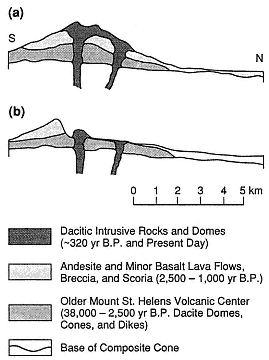
Fig. 7.14
North-south cross sections of Mount St. Helens in Washington illustrate changes resulting from
the sector collapse and eruption of May 18, 1980, and subsequent dome growth. (a) A pre-eruption
cross section shows: (1) the older volcanic center, consisting of nested dacite domes, pyroclastic
flows, and mudflow breccias, which is cut by dikes; (2) andesitic and basaltic lava flows interbedded
with volcanic breccias and scoria; and (3) several dacitic domes: the summit dome, erupted 370 years
BP and the north slope dome erupted between 180 and 138 years ago). (b) A posteruption cross
section through the crater caused by sector collapse and the avalanche that preceded the most
explosive phase of the eruption. Present-day development of the growing dacite dome within the
crater is indicated. (Adapted from Voight et al ., 1981.)
rocks to form 1- to 2-km-diameter aureoles of hydro-thermal alteration around intrusions. Surface indicators of the hydrothermal system include andesitic, dacitic, or rhyolitic vents; fumaroles and sulfur deposits at the summit; collapse craters; hydrothermal explosion breccias; and a long cone history (>100,000 years).
Sillitoe's (1973) general model for composite cones and associated intrusive rocks was based on his observations of copper-porphyry deposits in the Andes of Chile and northern Argentina—a region where volcanoes overlie thick crust. The hydrothermal systems responsible for deposition of the porphyry copper deposits were established late in the history of the composite cones; subsequently, only dacitic or rhyolitic magmas were intruded and erupted. Observations of these systems at various levels exposed by erosion (or in mine shafts) indicate that large granodiorite plutons are present at shallow depths (~4 km below the summits) during late-stage activity (Fig. 7.16). The chief distinction between these volcanoes and those in island arcs is the difference in crustal thicknesses beneath them; processes within the thick crust of the Andean altiplano ultimately produce large silicic magma bodies that are emplaced at shallow depths.
Giggenbach (1989) developed a similar model for an Andean composite cone, the young, active volcano of Nevado del Ruiz in Colombia. His model was based upon the analysis of gases and waters from Ruiz' fumaroles and hot springs. According to Giggenbach's interpretation, there is a broad mass of crystallizing, degassing magma and rock from ~3 km to >15 km below the summit (the volcano's elevation is 5389 m). Intrusions below Ruiz may be surrounded by aureoles of vapor, a mixture of brine and vapor, and brine. The vapor and vapor-plus-brine aureoles are of the same shape and
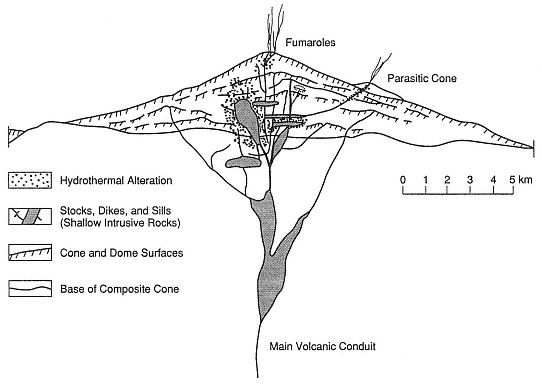
Fig. 7.15
Simplified cross section through a "nearly extinct" mature composite cone. This model was developed
by Branch (1976), who studied many composite cones in Papua New Guinea to determine their
potential for volcanogenic ore bodies. The model is based upon studies of
volcanoes in various evolutionary stages.
areal extent as the zone of hydrothermal alteration within a mature andean composite cone, which was proposed by Sillitoe (1973). The model for Nevado del Ruiz has not yet been tested by drilling.
Well-Mapped Examples of Eroded Composite Cones
Composite cones formed during Tertiary time usually have been deeply eroded and their interior structures are exposed. However, these cones are still sufficiently preserved to allow interpretation of the relationship between rock types and the structural framework. The cones we discuss here were chosen because their carefully executed three-dimensional maps with cross sections provide excellent examples of older composite cones.
Broken Top Volcano
At Broken Top volcano, in the southern Cascade Range of Oregon in the U.S., dissection by glaciation has provided a clear view of its interior workings (Crowe and Nolf, 1977). The cone consists of interbedded lava flows, laharic breccias, and other pyroclastic deposits illustrated in Fig. 7.17. The tall cone could not have remained standing without its complex internal framework, which is composed of dikes, sills, and small plutons. Early phases of cone construction were followed by collapse and the formation of a small summit caldera. After further cone construction, the edifice was intruded by plugs, radial and concentric dikes, and sills. Intrusions at Broken Top volcano constitute 5 to 20% of the cone volume.
Tieton Volcano
The Miocene-age Tieton Volcano of Washington has been deeply eroded, exposing radial dike swarms and plugs (Swanson, 1966). Originally, Tieton volcano had a basal diameter of ~11 km and a height of 2.4 km. The composite cone, made up of interbedded breccias, pyroclastic deposits, and block lava flows, overlies a shield composed of andesitic lavas. Within the southern, exposed half of the volcano, 200 dikes form a radial swarm; individual dikes are 2 to 6 m thick, are steeply dipping (70 to 90°), and are mostly andesitic. These dikes would have provided a substantial heat source if they were intruded over a fairly short period of time, but not if they were intruded piecemeal over tens of thousands of years. Such dikes
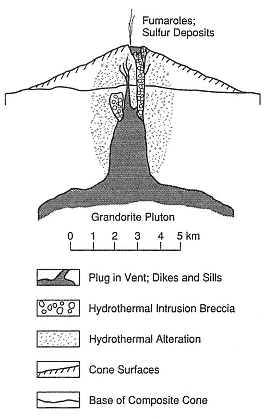
Fig. 7.16
This idealized cross section of a composite
cone and associated intrusive rocks is based
on observations of copper-porphyry
deposits in the Andes of Chile and northern
Argentina. Hydrothermal systems
responsible for the porphyry copper
deposits were established late in the history
of the composite cone; the intrusion and
eruption of rhyolitic magmas followed. In
this model, which is based on a region
with thick crust, the vertical and
horizontal scales are the same.
(After Sillitoe, 1973.)
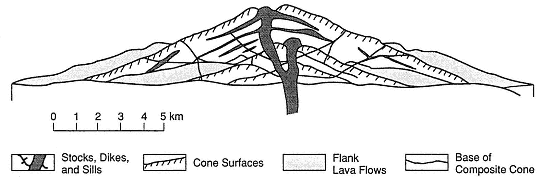
Fig. 7.17
A cross section of Broken Top volcano in the southern Cascade Range of Oregon,
in the U.S. The cone consists of interbedded lava flows, laharic breccias, and other pyroclastic
deposits. The cone could not have supported itself without the complex internal framework of
dikes, sills, and small plutons—all of which give tall composite cones their stability.
(Adapted from Crowe and Nolf, 1977.)
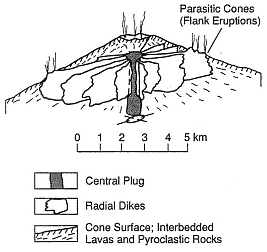
Fig. 7.18
Diagram showing typical dike patterns radial
to the central conduit of a composite cone
and the parasitic cones on the volcano
flanks. The dike swarms follow the trend
of maximum horizontal compression.
(Adapted from Nakamura et al., 1977.)
also act as barriers to groundwater flow and thus can "compartmentalize" aquifers or parts of a hydrothermal system.
Volcanoes of the Aleutian Arc and Alaskan Peninsula
In their study of arc volcanoes of the Aleutian Islands and Alaskan Peninsula, Nakamura et al . (1977) mapped radial dike patterns and parasitic cones on volcano flanks. They concluded that dikes and flank vents on composite cones form elongate swarms in regions under compression and that the swarms follow the trend of the maximum horizontal compression (Fig. 7.18). Inference of the location and dimensions of such dike swarms must be made when siting exploration wells because although they provide the heat source, they also may act as barriers to groundwater flow (see Chapter 6).
Nakamura's observations can be applied to many composite cones that exhibit sector collapse. This type of collapse may occur parallel to the dilational stress within the volcano when the volcano's flanks are forced outward (Siebert, 1984). However, it is more likely that the collapse process is related to the shape of the dike-sill complex within the cone. Composite cones devel-
oped in regions with homogeneous stress are supported by a radial framework of dikes and sills, whereas cones developed in regions strongly influenced by the regional stress regime have dikes that are located mostly along a line parallel to the maximum horizontal compression. Parallel dike systems support only the part of the volcano below a line of parasitic vents; unsupported flanks, with only rare dikes or sills, are subject to sector collapse.
Summer Coon Volcano
The mid-Tertiary-age Summer Coon composite cone in Colorado, mapped by Lipman (1968), is a deeply dissected cone in which the nearly circular complex of stocks is exposed, as are the radial dikes shown in Fig. 7.19. These silicic dikes are as much as 4.8 km long and 60 m wide. Remnants of the cone consist of interbedded tuff-breccias and lava flows of mafic to intermediate
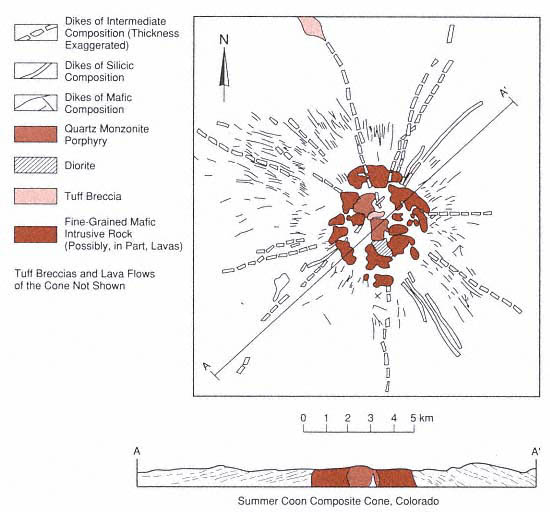
Fig. 7.19
Geologic map and cross section of the mid-Tertiary-age Summer Coon
composite cone in Colorado. This is a deeply dissected cone in which both the
nearly circular complex of stocks and the radial dikes are exposed.
(Adapted from Lipman, 1968.)
compositions. The symmetry of the dike complex reflects fracturing and dike emplacement controlled by stress around the central pluton or plutons and little superimposed tectonic control. The volcano's location on thick continental crust makes the Summer Coon volcano model more similar to volcanoes of the Andean altiplano than to the island arc volcanoes.
Mount St. Helens
Major structural changes to Mount St. Helens in Washington were caused by the sector collapse and eruption on May 18, 1980, in addition to subsequent dome growth. Before the eruption, Mt. Saint Helens consisted of (1) an older volcanic center with nested dacite domes, pyroclastic flows, and mudflow breccias, which was cut by dikes (Fig. 7.13); (2) andesitic and basaltic lava flows interbedded with volcanic breccias and scoria; and (3) dacitic domes (the 370-year BP summit dome and the 180- to 138-year BP domes of the north slope).
After the sector collapse and an avalanche that preceded the most explosive phase of the eruption at Mount St. Helens, an ampitheater-shaped crater remained. The dacite dome within the crater continues to grow (Voight et al ., 1981).
A Facies Model
Ruapehu
Ruapehu volcano in New Zealand, elevation 2797 m, was constructed by four major cone-building phases over the last 250,000 years. The 110-km3 composite cone is characterized by many abrupt lateral and vertical facies changes (Hackett and Houghton, 1989).
The central and flank vents, aligned along a north-northeast-trending lineament, consist of plug- and dome-like intrusions, thin lava flows, welded pyroclastic fall deposits, and vent breccias. Most of the vent areas have been hydrothermally altered. Proximal (near-vent) facies are made up of mostly block lava flows and lesser ashfall and laharic breccia deposits. The near-vent cones were intruded by numerous thin (0.5- to 5-m wide) dikes that are compositionally identical to adjacent lavas.
Hackett and Houghton concluded that the distal (ring plain) facies consists of interbedded epiclastic volcanic deposits that are interbedded with ashfall deposits and fluvially reworked avalanche or volcanic mudflows. This plain forms a 6- to 15-km-wide girdle around the composite cone, which is formed by overlapping alluvial fans. Hummocky mounds on the plain are part of a sector collapse avalanche deposit.
Hackett and Houghton also determined that the cones at Ruapehu are mostly constructed of lavas and domes and that pyroclastic and epiclastic materials are found mostly on the ring plain.
A Model Based on Heat Flow Measurements
Hakone Volcano
Iriyama and Oki (1978) measured temperature profiles in Hakone Volcano, Japan. However, the model was for a composite cone that grew within a small caldera. Hydrothermal activity is most intense in the eastern half of the caldera—probably because recharged groundwater from the lake in the western half of the caldera flows toward sea level in the east (Fig. 7.20). The thermal regime is most likely related to the caldera and not to the overlying composite cone.
Composite Cone Geothermal Systems
As discussed earlier in this chapter, many of the geothermal systems that were believed to be part of composite cones are actually located below the mature cones or are linked to other structures such as calderas and recent extensional faulting. Within the
Cascade Range of the U.S., there are few surface manifestations of hydrothermal activity at young composite cones (Duffield, 1983). Basaltic and andesitic volcanoes are generally not underlain by large, shallow crustal magma reservoirs. Only the most mature of these cones contain known geothermal reservoirs: for example, Crater Lake and Newberry volcanoes in Oregon, where there are young, voluminous, silicic magmas and small calderas.
For many composite cones, the traditional exploration methods such as measuring shallow heat flow, examining surface manifestations (hot springs, fumaroles, acid alteration, etc.), and evaluating electrical geophysical data are not successful (LaFleur, 1983; Wright and Ward, 1983). In most cases, high infiltration rates for rainfall and snowmelt near volcano summits can hydrologically mask any geothermal systems because the summit and slopes are saturated with cold water to depths of 500 m to 1 km. Within the Cascade Range of the western U.S., this phenomenon is known as the "rain curtain" (Swanberg et al ., 1988). Groundwater heated by plutons below Quaternary-age volcanoes of the Oregon Cascades flows laterally into the upper zones of older volcanic rocks on the flanks of the volcanic chain. The result is an area of near-zero shallow conductive heat flow around the young volcano as well as shallow high thermal gradients in the older rocks (Ingebritsen et al ., 1989). In this environment, the only effective exploration/evaluation methods are (a) a search for indirect clues such as youthful silicic tuffs and lavas and phreatic craters, (b) the qualitative maturity index (Fig. 7.6), and (c) intermediate to deep thermal gradient holes.
Proven (Drilled) Geothermal Fields
Meager Mountain
Meager Mountain in British Columbia, Canada, with a volume of 70 km3 and a cone height of 1500 m, comprises nine overlapping andesitic and dacitic volcanic assemblages that range from late Pliocene to 2440 years BP (Souther, 1985). Its multiple vents are aligned along a north-south trend. The volcano overlies a high-relief surface of older plutonic and metamorphic rocks (late Mesozoic to early Tertiary Coast Plutonic complex and late Miocene epizonal plutons). The older rocks consist of andesitic flows and volcanic breccias, and the younger rocks are dacitic flows and domes, ashfall deposits, and ignimbrites.
Warm springs of ~60°C issue from faults within the basement rocks at the base of the volcano. Much of the anomaly is masked by near-surface meteoritic water. Fifteen
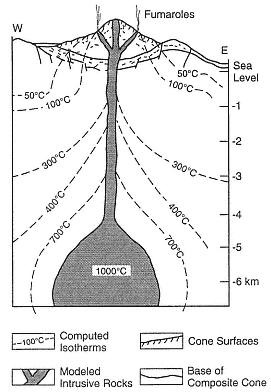
Fig. 7.20
This computed temperature profile of
Hakone volcano in Japan was created for a
composite cone located within a small caldera.
(Adapted from Iriyama and Oki, 1978.)
coreholes were drilled on the northern and southern flanks of the volcano (Adams et al ., 1985). Argillic alteration of the basement rocks was detected on the northern slope, but no hydrothermal fluids were found. Below the southern slopes, a plume of hot water (~200°C) rises along north-south-trending faults and along fractures within basement rocks; 233 to 264°C fluids were encountered at depths of 3 to 3.5 km in fractured plutonic rocks.
The hydrothermal resource is fracture-dominated and follows the Meager Creek fault zone, where the host rocks have low permeability and porosity. It is likely that the fluids are heated by subvolcanic intrusive rocks, but the amount of thermal energy contributed by cooling dikes and plugs is not known. The system may be related to deep circulation of fluids along the Meager Creek fault zone. Souther (1985) reported on two drilled reservoirs; the shallow, low-temperature (<140°C) reservoir has been clogged with authigenic minerals and forms a barrier for deeper, hotter fluids; the deeper reservoir, in fractured basement rocks, has temperatures >200°C.
Momotombo
The Nicaraguan Depression in Central America, which lies parallel to a northwest-southeast-trending plate boundary, encompasses most of the Quaternary-to-historical volcanic fields of Nicaragua. Momotombo Volcano, located on the northwestern shore of Lago de Managua, is a 1258-m-high symmetrical cone, with a volume of 12 km3 (Fig. 7.21 and cover photo). Momotombo is a submature composite cone within a northwest-southeast-trending graben and adjacent to a 4-km-diameter caldera. The somma ridge of an undated but older cone is located ~900 m above the base of Momotombo. The youngest rocks here are andesitic lavas that were erupted in 1905 AD (Mooser et al ., 1958).
Surface geothermal manifestations include active fumaroles in the summit crater and hydrothermally altered ground over a 4 km2 area along the southern slope of the volcano. This altered ground follows hydrothermally active, northwest-trending fractures (Figs. 7.21 and 7.22). Drillholes up to several kilometers deep were sited in interbedded andesitic lavas, welded tuffs and ashes, basaltic lavas and ashes, and dacitic lava; the most common rock type is andesitic lithic tuff (Moore et al ., 1982). Some of the rocks have been interpreted as phreatomagmatic—which is certainly possible because Momotombo sits on the shore of Lake Managua.
Maps of the alteration aureoles suggest structural control and fracture permeability within the reservoir and have provided an excellent basis for siting both exploration and production drillholes. Most of the wells with high permeability are within 100 m of a high-angle reverse fault and a right-lateral strike-slip fault. Some of the fractures show signs of secondary brecciation and may have formed by hydraulic fracturing (Moore et al ., 1982). Drilling has determined that there is good correspondence between surface and subsurface structure. The highest temperatures (225°C) are within a lens-shaped reservoir at depths of 244 to 366 m. In 1979, six production wells supported a 30-MWe plant; Rowley (1982) estimated field capacity is 800 MWe , although that is an optimistic estimate.
Ahuachapán
The Ahuachapán geothermal field in El Salvador Central America is associated with the Laguna Verde volcanic complex, which consists of north-northeast-trending lines of craters and small cones. The 500- to 600-m-diameter craters at the summit of these composite cones may be phreatomagmatic. These volcanoes are composed of interbedded andesitic tuffs, lavas, and agglomerates that range from Pliocene to Holocene (a very general estimate by Romagnoli et al ., 1976).
Also associated with the Laguna Verde volcanic complex and north-northwest-trending
normal faults are two major hot spring areas and three fumarolic fields with temperatures as high as 123°C. The geothermal wells located in this area are producing fluids from interbedded pyroclastic rocks and lavas with temperatures of 228°C at a depth of 900 m. Production wells have been drilled to average depths of ~800 m. The fumaroles, hot springs, and most of the drillholes are located near intersections between north-northwest-trending faults and east-northeast-trending faults. Two 30-MWe - and one 35-MWe -capacity electrical generating plants were completed by 1987.
Ohnuma
The Ohnuma geothermal field of Japan is located on the flank of Hachimantai Volcano, an 800-m-high, flat-topped lava shield composed of mafic andesitic lava flows (Kuno, 1962). This immature composite cone, where lavas range from 0.5 to 1.0 Ma, has a volume of ~13 km3 .
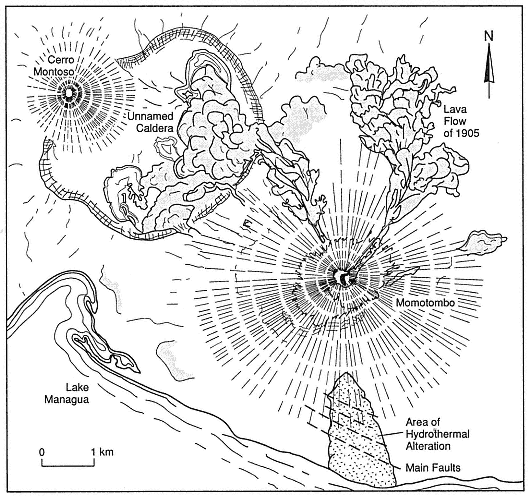
Fig. 7.21
Map of Momotombo Volcano in Nicaragua shows the main faults and
areas of alteration, which are marked with a stippled pattern.
(Adapted from Mooser et al ., 1958; Moore et al ., 1982.)
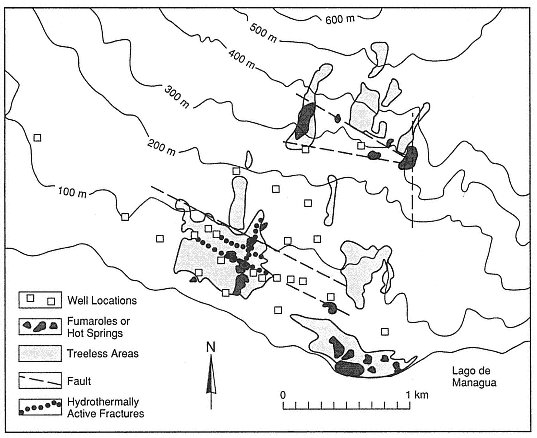
Fig. 7.22
The Momotombo geothermal field is located on the southern slopes of
Momotombo volcano and on the edge of Lake Managua.
(Adapted from Moore et al., 1982.)
Thermal areas marked by geysers, mudpots, fumaroles, and hot springs cover 5 km2 . The hydrothermal system may not be directly related to the composite cone, but rather to an older caldera and a north-south-trending graben. The reservoir is located within highly fractured dacitic and andesitic lavas and interbedded pyroclastic rocks (Nakamura et al ., 1981; Kimbara, 1986). Production wells have been drilled to depths of 1.3 to 1.7 km.
Matsukawa
The Matsukawa geothermal area of Japan is located between the Maru Mori and Iwate volcanoes. The area's largest cone is 800 m high and the volcanic complex has an approximate volume of 45 km3 . This complex includes composite cones, a dome, and a 1.8- by 3.0-km-diameter summit caldera. Kuno (1962) reported that its lava flows and volcanic breccias consist of augite-hypersthene andesite (in the older part of the cone) and olivine andesite (in the main cone). The summit crater contains fumaroles and small areas of acid alteration. This complex is believed to be of Pleistocene and Holocene age.
The geothermal area is marked by a 0.5- to 1- by 7.0-km east-northeast-trending zone of argillization, silicification, and hot springs. The zone overlies a geothermal reservoir at a depth of 1.0 to 1.2 km in fractured welded tuff and shale; there is a caprock of welded tuff (Nakamura et al ., 1981; Kimbara, 1986).
Because the geothermal system is located on the rim of a caldera, it may be only partly related to the cone.
Tamagawa Spa
The Tamagawa Spa of Japan is a group of acidic hot springs located at the western foot of Mount Yake (Yakeyama). Mount Yake is a 750-m-high mature composite cone with a volume of 10 km3 (Kuno, 1962); the cone's 600-m-diameter summit crater contains a small dome and four small explosion craters. There is also a parasitic dome on the southern flank of the main cone. Mount Yake is composed of mostly hypersthene-olivine andesitic lava flows and pyroclastic rocks, and the domes are andesitic. The most recent eruption products are Holocene. This cone may overlie the rim of an older caldera and the hydrothermal system could be associated with that caldera.
The geothermal area (spa) comprises lines of fumaroles, hot springs, and deposits of silica sinter, all of which are oriented east-west and north-south. The springs have temperatures up to 98°C and pHs of <1.2. The main geothermal area is located in a depression that has been interpreted as both a phreatic crater and a landslide. The reservoir is located within fractured Tertiary-age tuff, shale, and andesitic lava (Nakamura et al ., 1981).
Bouillante
The volcanoes of Guadeloupe, in the Lesser Antilles, include composite cones and domes and are located along the north-south-trending spine of Basse-Terre (the high, volcanic portion of the island). Soufrière de Guadeloupe is a dacitic dome with a summit 1467 m above sea level and is the site of historic eruptions that included explosive phreatic activity 1976 to 1977 AD. Eruptions of Soufrière de Guadeloupe have produced phreatic deposits, pyroclastic fall deposits, ignimbrites (mostly nonwelded), andesitic lavas, laharic breccias, and dacite domes. Vatin-Perignon et al . (1984) documented ages from 0.3 Ma to the present.
The summit region of Soufrière, site of the youngest cones and domes, is cut by northwest- and northeast-trending faults, along which there are surface manifestations of the geothermal system such as summit fumaroles and large areas of acid alteration. Hot springs are located around the base of the most recent dacite dome, which was recently modified by phreatic eruptions (Heiken et al ., 1980).
A commercially developed hydrothermal system is located on the island's west coast along a northwest-trending fault and a line of small cones that extends from Soufrière de Guadeloupe down to the coastline (Fig. 7.23). At Bouillante, there are areas of hydrothermal alteration and silicification as well as a 98°C spring. The geothermal reservoir, located within Tertiary tuffs and andesitic lava flows, has both fault (fracture) and formation permeability. Temperatures at a depth of 500 m are >240°C (Demians et al ., 1972; Vatin-Perignon et al ., 1984). Epidote appears in rocks below 100 to 300 m.
Kawah Kamodjang
The fumarole field of Kawah Kamodjang in Indonesia is located in western Java, where its specific association with a composite cone or cones is not clear. The nearby volcanoes of Rakutak, Chihara, Danou, Pangkaban, Gandapura, Masagit, and Guntur are part of a 15-km-long, 4- to 5-km-wide volcanic chain, which is parallel to a graben that trends west-southwest and east-northeast; most faults strike N60°E. The highest cones have elevations of ~1500 m and are composed of well-bedded, massive pyroclastic rocks that are interbedded with thick andesitic flows (Neuman van Padang, 1951; Robert et al ., 1983). Robert et al . (1983) postulated that the geothermal area may be located within a small (2-km-diameter, 500-m-deep), poorly defined caldera.
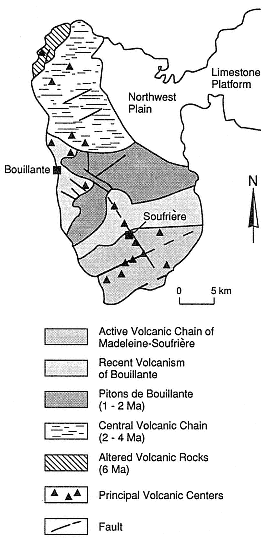
Fig. 7.23
Sketch geologic map of the western part of the
island of Guadeloupe (Basse-Terre) in the
Lesser Antilles. The Bouillante geothermal
area is located along northwest-trending
faults that extend downslope from the active
volcanic chain of Madeleine-Soufrière.
(Adapted from Gérard et al. 1981.)
Within the 2-km-wide depression is a 1200-m-long, 100- to 700-m-wide zone of fumaroles, mudpots, phreatic craters, and hot springs with temperatures of 80 to 105°C. Six production wells supplying a 30-MWe generator were drilled in small grabens within the larger graben. The drilled reservoir is located within hydrothermally altered, fractured tuffs and lavas, where temperatures reach 200 to 230°C, at a depth of 900 m (Robert et al ., 1983). The main caprocks are hydrothermally altered pyroclastic deposits.
Composite Cones with Possible Geothermal Potential—As Yet Unproven by Deep Drilling
Mount Shasta
Mount Shasta, one of the southernmost of the Cascade volcanoes, is located in northern California. With a volume of 350 km3 and an elevation of 3050 m, this mature composite cone is also the largest Cascade volcano. The summit crater, two older central vents, and a line of flank vents are located along north-south trends (Christiansen et al ., 1977). Large dacitic cones and flows have erupted from both summit and flank vents. A 450-km2 area northwest of the volcano is covered by a hummocky debris avalanche deposit, which Crandell et al . (1984) interpreted as a sector collapse of the main cone ~300,000 years BP.
The cone is made up of equal portions of lava flows and blocky pyroclastic debris, including pyroclastic flow deposits and volcanic mudflows (Christiansen et al ., 1977), most of which are composed of pyroxene-andesite and hornblende-bearing andesite. Basalt is found only below an altitude of 2100 m in cinder cones and small lava flows. Mount Shasta formed during the last 500,000 years, and four periods of cone-building activity have occurred during the last 250,000 years (Christiansen, 1985).
There are summit fumaroles and areas of intense local hydrothermal alteration on each of Mount Shasta's four main cones, but nowhere else. Theoretically, there should be a small hydrothermal system within or below this large composite cone; however, there is no evidence for such a system because of the effective shield established by high flux of cold water from rain and
snow on the mountain. To date, there has been no drilling on Mount Shasta to test for a geothermal system.
Mount Hood
Mount Hood, also in Oregon, is located within a broad graben that follows the summit of the north-south-trending topographic high, which is the backbone of the high Cascades of the Pacific Northwest. Mount Hood was an obvious target for geothermal exploration and research, and a cooperative effort was begun in 1976 by a consortium of federal and state agencies (Williams et al ., 1982).
Mount Hood is a 2200-m-high mature composite cone with an approximate volume of 188 km3 . Eruptions have occurred there over the past 700,000 years; the most recent was less than 200 years ago (Wise, 1969; Crandell and Meyer, 1977). Mount Hood is composed of interbedded thin lava flows and pyroclastic debris (ashfall deposits, ignimbrites, and laharic breccias). Little is known or has been inferred about intrusive rocks in Mount Hood (shown in cross section in Fig. 7.24) except for the "plug" dome that comprises andesitic and hornblende andesitic lava; peripheral cones are composed of basalt.
The only surface manifestations of a hydrothermal system on Mount Hood are summit fumaroles, at temperatures of 50 to 85°C, and areas of hydrothermal alteration surrounding the plug dome. Although 25 shallow wells have been drilled on the flanks of Mount Hood, no shallow magma chamber or large hydrothermal systems were detected; the shallow wells did not penetrate the near-surface outflow zone of cold groundwater.
The deepest geothermal gradient hole drilled at Mount Hood is located on the lower flanks of the volcano near faults in basalt flows that predate Mount Hood; the bottomhole temperature of this well is 120°C at a depth of 1.8 km (Priest, 1982). The thermal gradient of ~60°C/km in this corehole could be related to magmas in the cone, but it also can be explained solely by the high heat flow in this tectonically active area.
Because of the enormous terrain corrections required for analysis, and the presence of a cold groundwater shield below the slopes of Mount Hood, most traditional geophysical
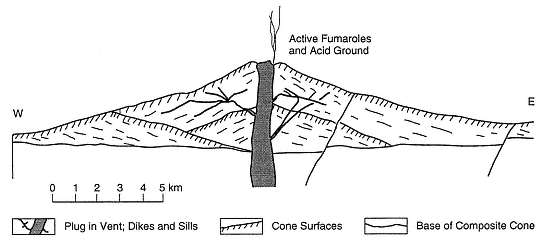
Fig. 7.24
Composite cross section of Mount Hood in Oregon.
(Based on work by Wise, 1968; Williams et al. , 1982; Priest, 1982.)
exploration methods did not reveal the presence or absence of a hydrothermal system. Shallow drilling penetrated some zones of warm water, but most drillholes never reached beyond the cold, near-surface groundwater. There may be a hydrothermal system below this large, young composite cone, but it has not yet been observed.
Mount Adams
Located east of the main Cascade trend, Mount Adams in Washington is a large (~200-km3 ) composite cone with an elevation of 3743 m. The composite cone is near the center of a basalt-to-rhyodacite volcanic field with more than 60 vents of Quaternary age. Flank vents, which occur at elevations of 2000 to 2500 m, are composed of mafic andesitic to dacitic lava flows and block-and-ash flows (Hildreth and Fierstein, 1985). Peripheral basaltic cinder cones and lava flows erupted on the lower flanks have a volume of 70 km3 . Volcanic activity began about 500,000 years ago, and the youngest eruptions took place 3500 years ago; most of the composite cone was constructed between 20,000 and 10,000 years ago.
There are summit fumaroles and warm springs near the base of the volcano. The breccia and scoria core of this cone has been severely altered by acid waters. The 4-km2 area of altered core is a source of avalanches and debris flows because of the gravitational instability of the clay alteration products (Hildreth and Fierstein, 1985).
On the basis of the petrology of erupted materials, Hildreth and Fierstein concluded that it is unlikely there is any significant magma reservoir within Mount Adams. High precipitation (3500 mm/year) makes the mountain an important recharge site and the extremely permeable carapace is saturated with cold water that does not remain long enough to be heated.
Mount Fuji
One of the Earth's most famous composite cones, Japan's Mount Fuji is composed of a group of 3 overlapping cones and 100 peripheral cones. Most of these vents are located along a north-northwest-south-southeast-trending line that is parallel to regional structures (Kuno, 1962; Tsuya et al ., 1981). The 1.5- × 0.7-km summit crater is 750 m deep. Older lavas at Fuji are olivine basalts, whereas the younger cones are composed of andesite and peripheral cones of olivine basalt. The cones comprise interbedded ashfall deposits, lahar deposits, ignimbrites, and lava flows. The complex is ~80,000 years old, and the most recent activity occurred in 1707 AD (Kuno, 1962).
All thermal anomalies on Fuji are masked by movement of shallow groundwater. Yuhara (1974) reported that each year 77% of the precipitation flows out at the foot of the cone and another ~20% is lost by evaporation. Tritium analyses have revealed that water emerging near the base is not old. Shallow aquifers follow scoria-lapilli beds and fractures in lava flows. Impermeable units are mudflow deposits and older basement rocks. Very little of the recharge water enters the volcano's interior to be heated. Mount Fuji has not been drilled for geothermal resource evaluation.
From Yuhara's (1974) observations at Fuji and Mount Yotei (also in Japan), it was determined that recharge into the hydrothermal system of a composite cone may not be adequate to maintain a hydrothermal system. Conditions necessary for adequate recharge will most likely depend upon the structural framework of the individual volcano, its maturity, fault patterns, and the degree of intrusion and hydraulic fracturing associated with dike-sill systems and small plutons. As described earlier, eroded, mature volcanoes in areas with thick continental crust expose large volumes of hydrothermally altered lava and pyroclastic rock.
Discussion
Techniques for the exploration and development of geothermal resources associated with composite cones are still in their
infancy. With the exception of ancient hydrothermal systems within composite cones, which have been studied chiefly in relation to their porphyry copper deposits, we have yet to determine if there are useful geothermal resources associated with most mature composite cones. In the earlier parts of this chapter, we proposed a general concept of composite cone maturity and its application to geothermal exploration and resource evaluation. To determine if reservoirs are present, intermediate to deep drilling will be required for a mature composite cone. Cold groundwater outflow within these cones often mask surface geothermal manifestations as well as the heat flow measurements in shallow drill-holes.
With good reason, successful geothermal sites have been drilled on the lower slopes of (or adjacent to) composite cones at sites where there are surface manifestations of a hydrothermal system. Healy (1976) points out that hot springs occur where heated meteoric water intersects the basal contact between the cone and older rocks. This phenomenon implies the presence of a convective plume within the cone—an inference confirmed by examination of hydrothermal alteration aureoles within eroded composite cones (for instance, see Sillitoe, 1973).
For many the examples cited here, it is not clear if the hydrothermal systems described are related to the nearby older volcanoes or to the overlying composite cones. In each of these instances there are still other interpretations. Several of the cones are sited along caldera rims, where they were formed by postcaldera eruptions, and nearly all of the cones are located along active faults. It is most likely that magma feeding the cones is contributing to the elevated heat flow in the area and that the high cones serve as major recharge areas. It is not clear if the plumbing (intrusions) within these cones is the major heat source for their hydrothermal systems.
In any case, all the tested geothermal systems associated with composite cones that were cited here have the following common features:
· all are located near or below the base or the lower slopes of the cone;
· all have ample surface manifestations of the hydrothermal system, including argillic and acid alteration, silica sinter deposits, hot springs, fumaroles, mudpots and/or geysers; and
· all have reservoirs in highly fractured basement rocks along active normal, reverse, and strike-slip faults.
In addition, some of the cones are located along the rims of underlying calderas and some have phreatic craters or phreatic breccia deposits (Fig. 7.25).
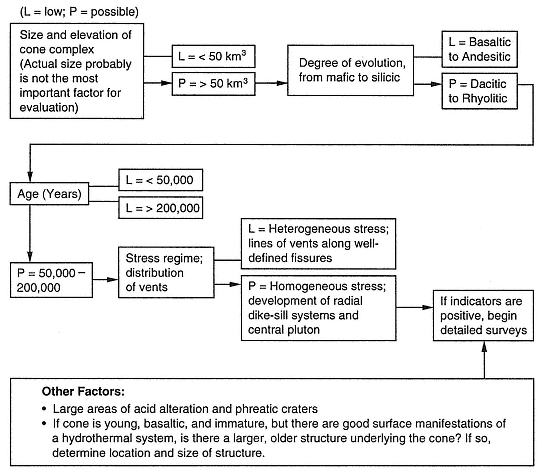
Fig. 7.25
Flow chart for exploration and evaluation of the geothermal potential of composite cones.