PART II—
BEHAVIOR AND LIFE HISTORY
Seven—
Juvenile Survivorship of Northern Elephant Seals
Burney J. Le Boeuf, Patricia Morris, and Joanne Reiter
ABSTRACT. The aim of this study was to determine the juvenile survivorship rate of northern elephant seals, Mirounga angustirostris , throughout the first four years of life and to assess the role of year, cohort, and condition at weaning on survival.
The study was conducted at Año Nuevo, California, during the years 1971–1978, a time when colony size was increasing. Pup mortality on the rookery prior to weaning was estimated from daily censuses during the breeding season. Juvenile survivorship was determined from resights of 8,362 individuals tagged on the rookery at weaning (about 30 days of age); systematic searches were conducted on the natal rookery as well as on neighboring rookeries. The effect of mass and length at weaning on juvenile survivorship to 1 and 2 years of age was determined from 734 weaned pups weighed and measured during the years 1978 and 1984–1988.
Mean percentage survival to age 1 was 36.8 ± 8.5; to age 2, 26.3 ± 6.3; to age 3, 19.4 ± 5.1; and to age 4, 16.3 ± 5.2. Most of the first-year mortality occurred at sea; on average, 31.5 ± 12.4% of the first-year mortality was due to neonate death on the rookery. Juvenile survivorship rates were lowest in El Niño years (1978, 1983, and 1986). As colony size increased fivefold over the study period, survivorship to age 1 did not change significantly, but survivorship to age 4 decreased significantly. No significant relationship was found between weanling mass and survival to 1 and 2 years of age. Survivorship to 1 year of age was positively correlated with standard length, but this relationship did not hold for survivorship to age 2.
The juvenile survivorship rate of seals from the Año Nuevo colony is too low to support the observed growth rate of the colony and of the population as a whole. Other California rookeries, such as San Miguel Island, must have significantly higher juvenile survivorship rates to account for the recent population increase. The causes of high juvenile mortality at sea are unknown; they do not appear to be related to condition at weaning, as reflected by weaning weight.
Survivorship and fertility schedules shape life history tactics (Stearns 1976, 1980) and provide vital demographic data for estimating population growth (Wilson and Bossert 1971). This chapter addresses juvenile survivorship in northern elephant seals, the percentage of individuals born that survive to each of the first four years of life. Our aim is to describe age-specific survival rates and age-specific mortality rates of seals born at Año Nuevo, California, over the last two decades when colony size was increasing and to examine the role of year, cohort, and condition at weaning—as reflected by weight, length, or an index of the two measures—on survival. Because juvenile survivorship is an important determinant of the growth or decline of a population, data presented here may elucidate the rapid growth of the northern elephant seal population over the last few decades as well as provide an instructive comparison with southern elephant seals, whose numbers are declining at several rookeries (Hindell, Slip, and Burton, this volume).
This chapter summarizes and augments data on juvenile survivorship of Año Nuevo-born seals presented in J. Reiter, N. L. Stinson, and B. J. Le Boeuf (1978), J. Reiter (1984), B. J. Le Boeuf and J. Reiter (1988), and B. J. Le Boeuf and J. Reiter (1991).
Background
Año Nuevo is a peripheral colony in the northern elephant seal range. Since breeding began here in 1961 (Radford, Orr, and Hubbs 1965), it has received immigrants from larger southern rookeries in southern California, San Miguel and San Nicolas islands. Throughout this period, the entire population has grown steadily (Stewart et al., this volume). Births at the Año Nuevo colony have increased at the rate of 14% per year, and annual pup production is now on the order of 2,000 pups. The growth, however, is due mainly to immigration from southern rookeries, for internal recruitment is too low for the colony to sustain itself (Le Boeuf and Reiter 1988).
Most females give birth for the first time at age 4 (range 3–6 years of age) and then give birth annually until death. Single pups are produced, nursed 25 to 28 days, and weaned abruptly when the mother returns to sea. The weaned pup fasts for 2½ months on the rookery while learning to swim and dive before going off on its first foraging trip (Reiter, Stinson, and Le Boeuf 1978).
Juveniles make two foraging trips per year, each lasting about five months (see fig. 13.1 in chap. 13). As a result, they appear on the rookery twice a year, in the spring and in the fall, each haul-out lasting about one month. At this time, they are identified and survival is estimated. The pattern changes when females begin giving birth. Consequently, sex differences
in survival begin to appear in year 3, partially a result of a sex difference in time spent at sea.
Methods
Pups born in the years 1971 to 1988 at Año Nuevo, California, were tagged shortly after weaning with one or two cattle ear tags in the interdigital webbing of the hind flippers (Le Boeuf and Peterson 1969). The number of seals tagged per year varied from 100 to 900. Survivors were identified when their tags were read at approximately weekly intervals on the island and the mainland resting and molting sites at Año Nuevo. Seals dispersing to other rookeries were identified and reported to us by H. Huber and W. Sydeman for Southeast Farallon Island, S. Allen for Point Reyes Headlands, and B. Stewart and R. DeLong for San Miguel and San Nicolas islands. Seals that stranded along the central California coast were reported to us by researchers at the California Marine Mammal Center.
From resights of tagged seals, we calculated life tables to age 4. The criterion for survival to age 1 was resighting the seal after the first trip to sea, when it was 9 to 10 months old in the fall or 15 to 17 months old in the spring. Survival to age 2, 3, and 4 was recorded similarly. In our experience, only slightly more juveniles are observed in the spring than in the preceding fall haul-out.
The survivorship data for each cohort and age class were adjusted to account for unrecognizable survivors that lost their tag identification. The proportion of animals that lost their tags varied from year to year because cohorts varied with respect to the proportion of single- and double-tagged individuals. Nearly all seals in the cohorts during the interval 1985–1988 were double tagged. For single-tagged seals, we assumed a tag loss rate of 11% per annum for the first two years of life and 6% per annum thereafter. The tag loss rate of double-tagged seals was assumed to be the rate of single tag loss squared, or 1.21% per annum for the first two years and 0.36% per annum thereafter. Tag loss estimates were based on the loss rate of single tags determined from double-tagged individuals (Reiter 1984)
The influence of weight, length, and a condition index on the probability of first-year survival was investigated. During 1978 and the years 1984–1988, 734 weaned pups were weighed and measured within a month of weaning. Weaning mass was estimated by back calculation based on known rates of mass lost per day (see equation in Appendix 10.1 of Deutsch et al., this volume). All pups that weighed less than 50 kg were excluded from the analysis. These were orphaned pups that did not suckle normally; most of them died on the rookery or stranded nearby shortly after going to sea. Standard length was measured in a straight line from tip of nose to tip of
|
tail above the dorsal surface. A condition index, ostensibly reflecting a pup's stored energy reserves, was calculated as mass divided by length.
Results
Age-specific Survival
Of the pups born during the years 1971–1988, the mean percentage that survived to age 1 was 36.8 ± 8.5; to age 2, 26.3 ± 6.3; to age 3, 19.4 ± 5.1; and to age 4, 16.3 ± 5.2. These data are presented as partial life tables in table 7.1 and figure 7.1. Age-specific survival varied widely over the years, with the following range of values being observed: 19.9–48.7% to age 1; 11.1–37.4% to age 2; 7.1–28.9% to age 3; and 5.0–26.5% to age 4. Survival rates were highest for the year 1971 and lowest for the year 1983 (fig. 7.2).
Age-specific Mortality
Age-specific mortality for the entire sample was highest during the first year of life, 63.2%, and then dropped steadily until reaching a low of 16% between 3 and 4 years of age (table 7.1, fig. 7.1).
Mortality on the Rookery and at Sea during the First Year
Over the study period, a mean of 31.5 ± 12.4% of the first-year mortality was due to neonate death on the rookery; the majority of the first-year mortality occurred at sea. The proportion of first-year mortality occurring on the rookery reached a high of 61% of pups born in 1983 due to the rookery being inundated by storm-whipped high surf at high tide during the peak
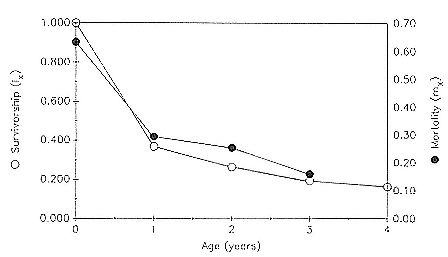
Fig. 7.1
Survivorship (lx ) and mortality (mx ) curves for the northern elephant seal at
Año Nuevo, California, during the years 1971–1988. Based on data in table 7.1.
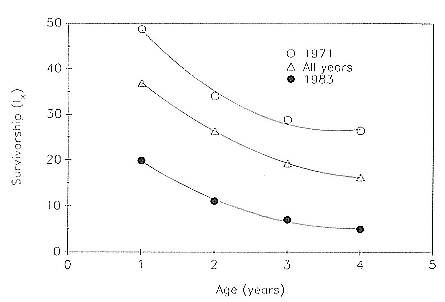
Fig. 7.2
Survivorship (lx ) curves for northern elephant seals from Año Nuevo, California,
during the best (1971) and worst (1983) years in the study period, 1971–1988,
and for all years combined.
pupping period (see also Le Boeuf and Condit 1983; Le Boeuf and Reiter 1991).
Survival Rates of Weanlings
For all years combined, the mean survival rate of weanlings over the periods at sea was 46.0 ± 7.7% (range = 35.0%–61.1%) to age 1; 32.8 ± 5.9% (range = 21.6–44.5%) to age 2; 24.2 ± 5.5% (range = 13.8–33.8%) to 3; and 20.3 ± 5.8% (range = 9.8–30.9%) to age 4.
The Effect of Year and Associated Conditions
The role of a specific year and associated conditions, such as weather and prey availability, is reflected by the annual survival rates of 1-, 2-, 3- and 4-year-olds in that year. For example, in 1981, survival to age 1 was 42.5 ± 4%; the survival rate of the 1980 cohort from age 1 to 2 during 1981 was 91.9%; the 1979 cohort survival from age 2 to 3 in 1981 was 96.0%; and the 1978 cohort survival from age 3 to 4 in 1981 was 97.5%, giving 1981 a mean score of 82.0%.
Calculated in this way, the mean score of all cohorts was 79.1 ± 3.6%. There were three years with mean scores one standard deviation or more above the mean, an indication that they were exceptionally good years: 1974 (84.6%), 1985 (83.3%), and 1980 (82.6%). There were three poor years: 1983 (72.7%), 1986 (74.0%), and 1978 (76.0%). All three poor years are categorized as El Niño years by oceanographers.
Cohort Variation
As the size of the Año Nuevo colony increased more than fivefold from 1971 to 1988 (an increase similar to that of the entire population; see Stewart et al., this volume), one might expect lower survivorship values in the later years due to increased competition for resources either on the rookery or on the foraging grounds. Pup mortality on the island prior to weaning increased from a low of 14.5% of pups born in 1971 to a high of 70% of pups born in 1983; preweaning pup mortality is density dependent, and there is a significant interaction with weather (Le Boeuf and Briggs 1977; Le Boeuf and Reiter 1991). There was no tendency for survivorship to age 1 to decrease with time and increasing density (fig. 7.3a); however, survivorship to age 4 decreased with time (the regression of y on x = 90.1 – 0.94x; r = 0.81).
An indication of the relative long-term strength of a cohort is the percentage decrease in the juvenile survival rate from year 1 to year 4. The percent decrease in survivorship from age 1 to age 4 increased over the study period (fig. 7.3b); that is, the mortality rate over the juvenile years increased with time.
The mean percentage decrease in survivorship from age 1 to 4 (fig. 7.3b)
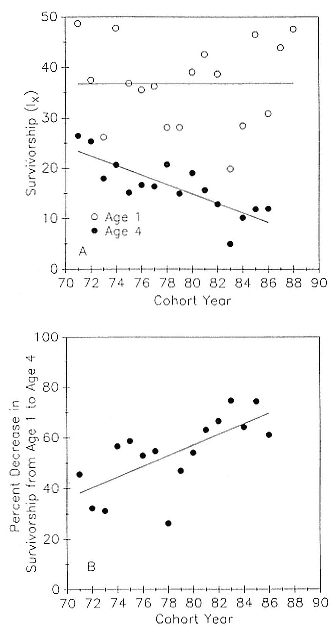
Fig. 7.3
(A) Survivorship to age 1 (open circles) and to age 4 (closed
circles) as a function of cohort year. The regression equation
for the latter is y = 90.1 – 0.94x; r = .81. (B) The percent
decrease in survivorship from age 1 to age 4 as a function
of cohort year. The regression equation is
y = –111 + 2.1x; r = .69.
for all 18 cohorts was –55.2 ± 14.1%. The three strongest cohorts, those with the lowest percentage decline over the three-year period, were 1978 (–26.2 ± 3%), 1973 (–31.3%), and 1972 (–32.3%). The three weakest cohorts were 1983 (–74.4%), 1985 (–74.4%), and 1988 (–66.9%).
The Effect of Weaning Mass, Size, and Condition on Age-specific Survival
Although the weight of weanlings varied greatly, with animals in the highest weight category being almost twice as large as those in the lowest weight category (fig. 7.4a), there was no significant relationship between mass at weaning and survival to 1 year of age (chi-square = 8.17, df = 8, p > .05) or to 2 years of age (chi-square = 6.83, df = 8, p > .05) (fig. 7.4b). Seals with the most common weights at weaning, in the range of 120 to 150 kg, had the lowest survivorship to year 1, 34.8 to 41.7%). However, these rates did not differ significantly from that of other weight categories. The extreme low and high weight categories incurred the greatest decline in survivorship from year 1 to year 2, but these differences, too, were not significantly different from the declines in other weight categories (chi-square = 8.53, df = 8, p > .05).
Survivorship to 1 year of age varied significantly as a function of standard length at weaning (chi-square = 12.9, df = 8, p < .05)—weanlings with the smallest standard lengths had the lowest survivorship—but this effect did not hold for survival to age 2 (fig. 7.5). Survivorship did not vary significantly with condition index.
Reasoning that high weight or great size might be advantageous in a poor year, we examined separately the year 1986, the only year in the weighed weanling sample that physical oceanographers categorize as an El Niño year. During El Niño years, foraging may be more difficult because of lower prey availability (Arntz, Pearcy, and Trillmich 1991). Using the same weight and length classes shown in figures 7.4 and 7.5, survivorship of the 1986 cohort did not vary significantly with any measure of condition.
Discussion
Northern elephant seals exhibit the most common survivorship curve in nature, Type III, which is characterized by a steep decline in survivorship at an early age (Wilson and Bossert 1971). Those that survive the juvenile period have a good chance of reaching maturity. The majority of young northern elephant seals that were born at Año Nuevo during this study (nearly two-thirds of them, on average) did not survive to 1 year of age, and only 20%, on average, lived to age 4. Most of the juvenile mortality to age 1 occurs at sea, and all mortality to age 2, 3, and 4 occurs at sea. White sharks, Carcharodon carcharias , and killer whales, Orcinus orca , are known predators on northern elephant seals (Ainley et al. 1981; Le Boeuf, Riedman,
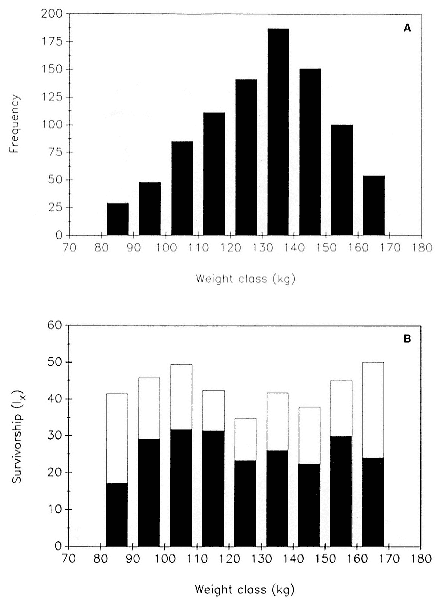
Fig. 7.4
(A) Frequency distribution of 734 northern elephant seal pups by weight class
at weaning. (B) Survivorship of northern elephant seal juveniles to age 1
(open bars) and to age 2 (closed bars) as a function of their weight class at
weaning. The sample is taken from Año Nuevo, California,
during the years 1978 and 1984–1988.
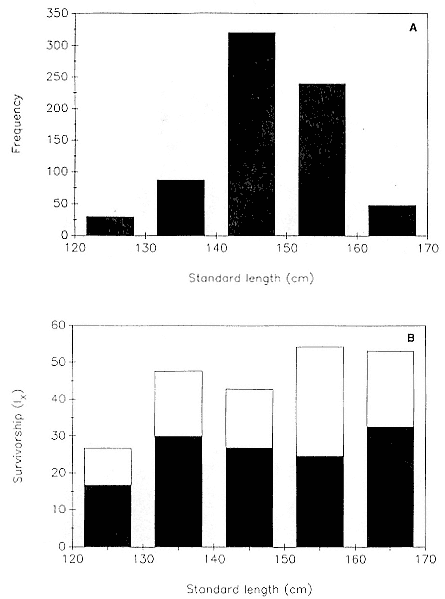
Fig. 7.5
(A) Frequency distribution of 734 northern elephant seal pups by standard length
class at weaning. (B) Survivorship of juveniles to age 1 (open bars) and to age 2
(closed bars) as a function of standard length at weaning.
and Keyes 1982; M. Pierson, pers. comm.), but the degree to which they account for the observed mortality rates is not clear.
In any case, the high juvenile mortality rate at Año Nuevo does not appear to be due to condition at weaning, insofar as condition is reflected by mass at weaning. Weaning weight (above 80 kg) was not correlated with survival to age 1 or to age 2. The fattest were not the fittest (see Sinervo et al. 1992). This is surprising given the large body of information on the importance of parental investment in enhancing individual reproductive success through the production of progeny (e.g., Clutton-Brock 1991). It remains to be determined whether mass at weaning is correlated with reproductive success.
Like other northern and southern elephant seal colonies (Huber, Beckham, and Nisbet 1991; Hindell 1990), cohort variation in juvenile survivorship at Año Nuevo was great, especially to age 1. Much of this variability was due to the effect of storms that occurred during the peak pupping period and caused high pup mortality on the rookery. A decrease in survivorship from age 1 to age 4 was evident over the course of the study period. This may have been due to increasing competition as the population grew or to interactions with fisheries, for example, high seas drift net fisheries in foraging areas. The cause of this decline may become clear as we begin to accumulate knowledge of the migratory paths and foraging areas of juveniles (Le Boeuf, this volume).
The juvenile mortality rate of Año Nuevo-born elephant seals is high relative to other well-studied large mammals such as Dall mountain sheep, Ovis dalli (Deevey 1947), and red deer, Cervus elephus (Clutton-Brock, Albon, and Guinness 1988), but strikingly similar to the low rates of male, relative to female, African lions, Panther leo , from the Serengeti population (Packer et al. 1988) and male vervet monkeys, Cercopithecus aethiops (Cheney et al. 1988).
Juvenile survivorship of northern elephant seals from the Año Nuevo colony is significantly lower than that of southern elephant seals from South Georgia, a large colony that was stable in numbers during the period 1951–1985 (Laws, this volume). T. S. McCann (1985) revised the life tables of R. M. Laws (1960) and estimated survivorship to age 1 as 60%, to age 2 as 51%, to age 3 as 43.5%, and to age 4 as 37.2% (we combined the sexes in his life tables for comparability). In comparison with this Southern Hemisphere rookery, juvenile survivorship at Año Nuevo was 42% lower to age 1 and 52% lower to age 4. Estimates of juvenile survivorship at Marion Island are similar to those at South Georgia, despite declines in number at the rate of 4.5% per year during the period 1974–1989 (Bester and Wilkinson, this volume).
Juvenile survivorship rates from Año Nuevo more closely resemble those of the declining southern elephant seal colony at Macquarie Island, studied
by R. Carrick and S. E. Ingham (1962) and M. A. Hindell (1990). Hindell (1991, this volume) estimated first-year survival at Macquarie Island during the 1950s as 44% (both sexes combined); however, from 1960 to 1965, first-year survival declined dramatically to 2%. Life table estimates of survival to each of the first four years of life (1x ), based on the entire study period, are 0.350, 0.298, 0.232, and 0.178, respectively. These values are similar to those reported in table 7.1 for Año Nuevo: 0.368, 0.263, 0.194, and 0.163.
These results are paradoxical, or at least do not appear to have a unitary explanation. Despite equally high juvenile survivorship rates, the Año Nuevo colony is increasing in number and the Macquarie Island colony is declining in number. Why is Marion Island declining in number despite a high juvenile survivorship rate? This state of affairs is not easily explained given current information. However, the following information is important for sorting out these incongruencies.
1. Año Nuevo may not be the ideal representative of an expanding colony for comparison with stable or declining colonies. The increase in the Año Nuevo colony, and other colonies like the Farallons at the northern boundary of the species' breeding range, is due mainly to dispersion and immigration from large rookeries in southern California, especially San Miguel Island; internal recruitment, alone, would lead to a decline in colony numbers (Le Boeuf and Reiter 1988; Huber, Beckham, and Nisbet 1991). San Miguel Island accounts for most of the growth of the entire northern elephant seal population (Stewart et al., this volume) and hence may best represent an expanding population. Presumably, juvenile survival rates at San Miguel Island are higher than those at Año Nuevo and perhaps even higher than those at South Georgia. Unfortunately, there are no data on juvenile survivorship for this rookery.
2. Considerable mixing occurs between colonies in the Northern Hemisphere. Consequently, the factors that make for growth of northern elephant seal colonies are more difficult to assess than those of southern elephant seal colonies, where immigration is rare or nonexistent (Burton 1985; Bester 1989; Gales, Adams, and Burton 1989; Hindell 1990) and growth depends ultimately on internal recruitment. For example, during the period 1969–1976, there was considerable dispersion of northern elephant seals among the seven extant rookeries (Bonnell et al. 1979). Movement was primarily in the northward direction and most prevalent during the first year of life. The majority of the movements represented permanent immigration. Southern California rookeries received immigrants from Mexican rookeries and sent out immigrants to northern California rookeries. There was
bidirectional exchange between rookeries separated by short distances, such as San Miguel and San Nicolas islands in southern California and Año Nuevo and the Farallons in central California.
3. Differences in adult female mortality might partially explain the different population trajectories of the expanding northern elephant seal population as compared to stable or declining colonies in the Southern Hemisphere. Adult female mortality is apparently lower at northern elephant seal rookeries such as Año Nuevo and the Farallons (Le Boeuf and Reiter 1988; Huber et al. 1991) than at the southern elephant seal rookery at Marion Island, where it is concluded that the colony decline is due mainly to high adult female mortality (Bester and Wilkinson, this volume). Natality of adult females does not appear to account for differences in growth of colonies since these rates are uniformly high at colonies in both hemispheres; that is, natality exceeds 85% of adult females (McCann 1985; Le Boeuf and Reiter 1988; Huber et al. 1991; Bester and Wilkinson, this volume). Longevity of females seems to be substantially greater at Año Nuevo than at Macquarie, but the data are from different eras and are not a fair comparison.
4. Differences in methodology may cause substantial variation in estimates of juvenile survivorship rates. For example, survivorship rates at Macquarie Island were determined by monitoring animals branded at weaning, while those at Año Nuevo and Marion Island were based on recovery of animals marked with cattle ear tags. Branding yields a permanent mark; tags are impermanent, and some of them are lost. When tags are used, the estimate of juvenile survivorship is affected by the estimate of tag loss. Additionally, tags are harder to see and read than brand marks. Consequently, tag loss is usually greater than estimated, leading to an underestimate of survivorship. That is, the survivorship rates based on tag resight data we have presented for the Año Nuevo colony are probably minimum estimates.
The pup mortality rate on the rookery before weaning, when the animals are marked, is another variable that affects estimates of juvenile survivorship. Deaths on the rookery are a component of the initial sample size. Neonate deaths on small rookeries like Año Nuevo can be counted directly or calculated with reasonable confidence from censuses of suckling and weaned pups. The mean preweaning pup mortality rate at Año Nuevo during the present study was 24.4 ± 10.7% of pups born. On large rookeries such as Macquarie Island, the preweaning pup mortality rate is assumed to be the rate observed in selected harems amenable to censusing. This rate was assumed to be 4.5% of pups born for South Georgia (McCann 1985)
and 5% of pups born for Macquarie Island (Hindell and Burton 1987). To what extent these different methodologies explain the wide disparity in preweaning pup mortality rates is not clear. A similar statement could be made about search effort, which, necessarily, varies with the size, terrain, and location of rookeries.
In summary, one can obtain reasonably accurate estimates of juvenile survivorship from small, expanding northern elephant seal colonies, such as Año Nuevo, but the extent to which they elucidate the role of juvenile survivorship in declining colonies is unclear. Indeed, Año Nuevo would be declining at a similar rate as Macquarie Island were it not for the influx of animals from San Miguel Island. It may be more important to document juvenile survivorship at San Miguel Island because its growth rate drives the growth of the population. But the task is made difficult by the sheer size of the colony and the need to estimate immigration and emigration rates.
Indeed, the most appropriate comparison, if not the easiest, is to compare the entire northern elephant seal population with that of either of the three main southern elephant seal populations defined by Laws (1960) as the South Georgia stock, the Kerguelen stock, and the Macquarie Island stock (see fig. 3.1, chap. 3). Like the northern elephant seal population, each southern stock is geographically isolated; animal movements between stocks are rare, and gene flow is limited (Gales, Adams, and Burton 1989). Animal movements within each population have an important effect on juvenile survivorship and female reproductive success and, ultimately, on population regulation.
References
Ainley, D. G., C. S. Strong, H. P. Huber, T. J. Lewis, and S. H. Morrell. 1981. Predation by sharks on pinnipeds at the Farallon Islands. Fishery Bulletin, U.S. 78: 941–945.
Arntz, W., W. G. Pearcy, and F. Trillmich. 1991. Biological consequences of the 1982–83 El Niño in the Eastern Pacific. In Pinnipeds and El Niño: Responses to Environmental Stress , ed. F. Trillmich and K. Ono, 22–24. Berlin: Springer Verlag.
Bester, M. N. 1989. Movements of southern elephant seals and subantarctic fur seals in relation to Marion Island. Marine Mammal Science 5: 257–265.
Bonnell, M. L., B. J. Le Boeuf, M. O. Pierson, D. H. Dettman, and G. D. Farrens. 1979. Summary Report, 1975–1978. Marine Mammal and Seabird Surveys of the Southern California Bight Area . III. Pinnipeds . Bureau of Land Management, Department of the Interior, Contract AA550-CT7-36.
Burton, H. R. 1985. Tagging studies of male southern elephant seals (Mirounga leonina L.) in the Vestfold Hills area, Antarctica, and some aspects of their behavior. In Sea Mammals of South Latitudes: Proceedings of a Symposium of the 52d ANZAAS Congress in Sydney—May 1982 , ed. L. K. Ling and M. M. Bryden, 19–30. Northfield: South Australian Museum.
Carrick, R., and S. E. Ingham. 1962. Studies on the southern elephant seal, Mirounga leonina (L.). V. Population dynamics and utilization. CSIRO Wildlife Research 7: 198–206.
Cheney, D. L., R. M. Seyfarth, S. J. Andelman, and P. C. Lee. 1988. Reproductive success in vervet monkeys. In Reproductive Success , ed. T. H. Clutton-Brock, 384–402. Chicago: University of Chicago Press.
Clutton-Brock, T. H. 1991. The Evolution of Parental Care . Princeton: Princeton University Press.
Clutton-Brock, T. H., S. D. Albon, and F. E. Guinness. 1988. Reproductive success in male and female red deer. In Reproductive Success , ed. T. H. Clutton-Brock, 325–343. Chicago: University of Chicago Press.
Deevey, E. S., Jr. 1947. Life tables for natural populations of animals. Quarterly Review of Biology 22: 263–314.
Gales, N. J., M. Adams, and H. R. Burton. 1989. Genetic relatedness of two populations of the southern elephant seal, M. leonina. Marine Mammal Science 5: 57–67.
Hindell, M. A. 1990. Population dynamics and diving-behaviour of a declining population of southern elephant seals. Ph.D. dissertation, University of Queensland, Australia.
———. 1991. Some life history parameters of a declining population of southern elephant seals, Mirounga leonina. Journal of Animal Ecology 60: 119–134.
Hindell, M. A., and H. R. Burton. 1987. Seasonal haul-out patterns of the southern elephant seal (Mirounga leonina ) at Macquarie Island. Journal of Zoology, London 213: 365–380.
Huber, H. R., C. Beckham, and J. Nisbet. 1991. Effects of the 1982–83 El Niño on northern elephant seals on the South Farallon Islands, California. In Pinnipeds and El Niño: Responses to Environmental Stress , ed. F. Trillmich and K.A. Ono, 219–233. Berlin: Spring Verlag.
Huber, H. R., A. C. Rovetta, L. A. Fry, and S. Johnston. 1991. Age-specific natality of northern elephant seals at the South Farallon Islands, California. Journal of Mammalogy 72: 525–534.
Laws, R. M. 1960. The southern elephant seal (Mirounga leonina Linn.) at South Georgia. Norsk Hvalfangst-Tidende 49: 466–476, 520–542.
Le Boeuf, B. J., and K. T. Briggs. 1977. The cost of living in a seal harem. Mammalia 41: 167–195.
Le Boeuf, B. J., and R. S. Condit. 1983. The high cost of living on the beach. Pacific Discovery 36: 12–14.
Le Boeuf, B. J., and R. S. Peterson. 1969. Social status and mating activity in elephant seals. Science 163: 91–93.
Le Boeuf, B. J., and J. Reiter. 1988. Lifetime reproductive success in northern elephant seals. In Reproductive Success , ed. T. H. Clutton-Brock, 344–362. Chicago: University of Chicago Press.
———. 1991. Biological effects associated with El Niño Southern Oscillation, 1982–83, on northern elephant seals breeding at Año Nuevo, California. In Pinnipeds and El Niño: Responses to Environmental Stress , ed. F. Trillmich and K. Ono, 206–218. Berlin: Springer Verlag.
Le Boeuf, B. J., M. Riedman, and R. S. Keyes. 1982. White shark predation on pin-
nipeds in California coastal waters. Fishery Bulletin 80: 891–895.
McCann, T. S. 1985. Size, status and demography of southern elephant seal (Mirounga leonina ) populations. In Sea Mammals of South Latitudes: Proceedings of a Symposium of the 52d ANZAAS Congress in Sydney—May 1982 , ed. J. K. Ling and M. M. Bryden, 1–17. Northfield: South Australian Museum.
Packer, C., L. Herbst, A. E. Pusey, J. D. Bygott, J. P. Hanby, S. J. Cairns, and M. B. Mulder. 1988. Reproductive success of lions. In Reproductive Success , ed. T. H. Clutton-Brock, 363–383. Chicago: University of Chicago Press.
Radford, K. W., R. T. Orr, and C. L. Hubbs. 1965. Reestablishment of the northern elephant seal, Mirounga angustirostris , off central California. Proceedings of the California Academy of Sciences 31: 601–612.
Reiter, J. 1984. Studies of female competition and reproductive success in the northern elephant seal. Ph.D. dissertation, University of California, Santa Cruz.
Reiter, J., N. L. Stinson, and B. J. Le Boeuf. 1978. Northern elephant seal development: The transition from weaning to nutritional independence. Behavioral Ecology and Sociobiology 3: 337–367.
Sinervo, B., P. Doughty, R. B. Huey, and K. Zamudio. 1992. Allometric engineering: A causal analysis of natural selection on offspring size. Science 258: 1927–1930.
Stearns, S. C. 1976. Life history tactics: A review of ideas. Quarterly Review of Biology 51: 3–47.
———. 1980. A new view of life-history evolution. Oikos 35: 266–281.
Wilson, E. O., and W. H. Bossert. 1971. A Primer of Population Biology . Stamford, Conn.: Sinauer Associates.
Eight—
Life History Strategies of Female Northern Elephant Seals
William J. Sydeman and Nadav Nur
ABSTRACT. We review the literature on variation in life history traits, evaluate evidence on the cost of reproduction, and investigate the significance and extent of individual differences in reproductive success for female northern elephant seals in California. Studies have demonstrated considerable variation in age of primiparity and natality rates and the consequences of this variation for individual fitness. Age at first breeding varies from 3 to 6, with a mode at age 4, and is influenced by socioecological factors. Natality is related to ecological factors and other life history traits. Studies generally support the concept of the cost of reproduction for female northern elephant seals. Costs of reproduction were expressed as a reduction in survivorship associated with age at first breeding and decreased future fecundity associated with either age at first breeding or natality; ultimate consequences for fitness have yet to be addressed. Future fecundity was negatively influenced by the number of prior reproductive bouts (an estimate of breeding intensity) for females that had attempted reproduction often for their ages. Individual variation in reproductive success was apparent. Some individual females had a history of being more successful than others at weaning their pups. The interactions among female quality and causes and consequences of variation for life history traits are considered. Additional study on natality in relation to fitness will serve to illuminate life history strategies that likely vary with individual quality.
Life history theory assumes that organisms cannot maximize survival and fecundity at the same time, because of constraints that may be physiological or ecological. As a result, higher reproductive effort will entail a cost in terms of subsequent survival or future reproductive success or both (Williams 1966; Stearns 1976; Schaffer and Rosenzweig 1977). The concept of a cost of reproduction has led to many studies on the apparent trade-off between current reproductive effort and the probability of future reproductive success (see reviews in Clutton-Brock 1988 and Nur 1990). Studies of
marine mammal life history traits in relation to the cost of reproduction are few because marking and tracking individuals through time is difficult. However, the northern elephant seal, Mirounga angustirostris , provides an excellent opportunity to examine life history parameters for pinnipeds because it is possible to track and monitor individuals throughout most of their reproductive lifetimes and to estimate fecundity and survivorship.
Our objectives here are (1) to review the literature on variation in reproductive traits that affect fitness for female northern elephant seals, (2) to evaluate evidence for a cost of reproduction, and (3) to examine variation in the pattern of successes and failures for individual females to determine the significance of factors acting between years within the same individual. The latter analysis is new and based on a long-term study conducted on the Farallon Islands, California; methods and results of this analysis will be detailed later. We focus our review on the adaptive significance of two life history traits: age at first breeding and natality rates. Natality is measured by the proportion of animals giving birth each year and varies with the frequency of intermittent reproduction or "skipping" by individual females. We evaluate life history traits, where possible, in regard to a cost of reproduction, expressed as either a reduction in survivorship or future fecundity; only three papers directly address the issue of a cost of reproduction in female northern elephant seals (Huber 1987; Reiter and Le Boeuf 1991; Sydeman et al. 1991). In reviewing the literature and the cost of reproduction, we reanalyze and reinterpret these original papers.
Life history traits and demographic parameters for northern elephant seals have been studied in detail on the Farallon Islands (38°N, 123°W) and Año Nuevo State Reserve (37°N, 121°W) in central California. Both of these colonies, located 90 km apart, are at the northern extreme of this species' distribution (see Stewart et al., this volume, for a range map). The Farallon Islands (Southeast Farallon Island, or SEFI) support 9 breeding groups or harems. At Año Nuevo (AN), there are about 15 harems on the island and the adjacent mainland. Colony numbers at the Farallones increased through 1983, before reaching a plateau (Huber 1987). The colony numbers continue to increase at AN (Stewart et al., this volume). Field methods were similar at each site and are detailed elsewhere. In our review, we consider both cross-sectional (Reiter, Panken, and Le Boeuf 1981; Huber et al. 1991; Sydeman et al. 1991) and longitudinal (Huber 1987; Le Boeuf and Reiter 1988, Le Boeuf, Condit, and Reiter 1989; Reiter and Le Boeuf 1991) studies from both the Farallon Islands and Año Nuevo.
Age at First Breeding
There is considerable variation in age at first breeding for female northern elephant seals. Modal age at primiparity was 4 years on SEFI (62.6% of
275 females; Sydeman et al. 1991) and AN (54% of 67 females; Le Boeuf and Reiter 1988). Age of primiparity varied from 3 to 8 for SEFI females and from 2 to 6 at AN. At AN, B. J. Le Boeuf and J. Reiter (1988) reported that 36% of the females first gave birth at 3 years of age, while at SEFI only 13% of first-time breeders were 3 years old (Sydeman et al. 1991). These values likely differ because Le Boeuf and Reiter studied the 1973 and 1974 cohorts from ANI, whereas Sydeman et al. reported data on females born from 1974 through 1985. Over the past two decades, each cohort has experienced differing social and ecological conditions that influence immature survival and recruitment probabilities (Huber et al. 1991; Le Boeuf, Morris, and Reiter, this volume). Colony size and competition for breeding space have changed dramatically during this period. H. R. Huber et al. (1991) showed that when SEFI colony growth slowed in the late 1970s and early 1980s, the proportion of 3-year-olds giving birth also decreased. However, Huber et al. also indicated that age at first breeding was delayed for cohorts affected by the 1982–1983 El Niño Southern Oscillation (ENSO). Thus, it is presently unclear whether changes in colony size or the ENSO explains changes in age at primiparity. Nevertheless, in comparison to the data set of Le Boeuf and Reiter, the SEFI data set analyzed by Sydeman et al. (1991) included many cohorts that were potentially influenced by the 1982–1983 ENSO and increased colony size. Consequently, average and modal age at primiparity for females first breeding in the 1980s was later than for females in the 1970s.
Intermittent Breeding and Natality
We define intermittent breeding, or "skipping," as forgoing pupping in any year during an animal's breeding lifetime, for example, after a female has become parturient but before she dies or ceases to reproduce altogether due to senescence. It is possible that a "skip" could be confused with an animal giving birth at another colony, or giving birth and losing the pup before being observed. Although skipping may be easily confused with poor coverage, we believe that the intensive research programs on northern elephant seals make this scenario unlikely. At present, all seal rookeries in central and southern California are under study. At AN and SEFI, weather permitting, individual subcolonies are visited daily by researchers, tagged cows are recorded, and animals are temporarily marked with hair dye to facilitate individual identification. At Point Reyes, only 25 km from SEFI, coverage is less frequent (about once per week), but for the past five years most tagged animals have been identified (S. G. Allen, pers. comm.). Through a cooperative exchange of information, movement of females between AN and SEFI and between SEFI and Point Reyes has been documented, but in general, most females are extremely site tenacious once they begin pupping
at a particular site. Emigration from a site followed by immigration back to the original location has not been observed. Consequently, we believe that case records of intermittent reproduction are real, not errors associated with poor coverage of breeding areas.
On SEFI, animals that forgo breeding in one year and are confirmed alive and breeding in subsequent years (i.e., "skippers") are seen during the winter breeding season (6% of all those observed to breed intermittently) or fall haul-out (15%) period, but most are seen during spring molt or in a subsequent breeding season (79%; Huber et al. 1991). Huber et al. (1991) demonstrated that skipping is fairly regular among multiparous females, although it is apparently more common for young, 4- to 5-year-old, animals (Huber 1987). Natality of females, 5 to 10 years old, averaged 80% among years, indicating that approximately 20% of the SEFI females do not pup in any given year. On AN, however, Le Boeuf and Reiter (1988) reported that age-specific natality varied little after first reproduction and that 97% of the females present on the rookery during the breeding season each year gave birth (compared with 94% on SEFI).
Whether skipping is associated with a life history strategy or a constraint imposed by ecological or social conditions is open to speculation. Intermittent breeding (natality) also varies with ecological effects, such as ENSO, and life history traits such as age at first breeding. Huber et al. (1991) documented that natality rates on SEFI were lowest in the years following the 1982–1983 ENSO. Similar to Huber et al.'s findings on age at first breeding in relation to ENSO, reduced food resources in 1983 may have had a negative effect on female body condition, which, in turn, affected natality rates in 1984 and 1985. Overall natality rates in 1986 recovered to pre-ENSO levels, indicating a short-term effect. Huber (1987) found that females who began breeding at an early age were more likely to skip breeding in subsequent years than females that deferred reproduction.
The Cost of Reproduction
Survival and Age at First Breeding
Reiter and Le Boeuf (1991) studied survivorship to age 8 of female northern elephant seals that first bred at age 3 (P3 group) versus females that deferred breeding until age 4 (P4 group). Females who bred at age 3 had lower survival to age 4 than females that did not breed at age 3. This result provides strong evidence for the cost of reproduction among the youngest parous animals. Reiter and Le Boeuf attributed this result to the immediate energetic cost of reproduction, which is greater in young primiparous females who are still growing.
Survival of P3 females between the ages of 4 and 8 was lower than the survival of P4 females to age 8 (26.8% vs. 37.0%). Using a linear regression
analysis on grouped data, Reiter and Le Boeuf (1991) reported a significant difference in the slopes of these survival curves. For a variety of reasons, however, we believe this approach was questionable. On statistical grounds, the linear regression used did not take into account differences in sample size for each age, heteroscedasticity in survival variance, and lack of independence between observations and assumes that residual errors are normally distributed. On biological grounds, female survival to the year after first breeding was included for the P4 group but not for the P3 group. This is an important distinction because the survival of P3 females to the year following first breeding (78.4%) was actually greater than the survival of P4 females to the subsequent year (74.3%). Omitting these data biases the results in favor of higher survival for the P4 group. Notwithstanding these difficulties, the data presented by Reiter and Le Boeuf are critical to understanding the evolution of life history patterns.
We have reanalyzed the survival data presented by Reiter and Le Boeuf (1991) using a form of survival analysis, the Cox Proportional Hazards Model (CPHM; Kalbfleisch and Prentice 1980). This technique has become very popular among biostatisticians, in part because it does not assume a normal distribution in the response variable (survival), nor is it unduly sensitive to small sample sizes and influential data points. Using the CPHM, we considered survival for P3 females from age 3 to 7 and P4 females from age 4 to 8, a four-year comparison for both groups including the year after first breeding. For these data, the CPHM did not indicate a significant difference (LRS = 2.84, p = .092), although perhaps with additional data the trend would be significant. Other possible comparisons (e.g., age 4 to 8 for both groups) yielded higher p-values (p > .10). We believe that the difference between our conclusions about statistical significance and those of Reiter and Le Boeuf (1991) reflect the fact that soon after first breeding, when sample sizes were large, survivorship to the first year after breeding was actually higher for the P3 group (78.4% for P3 vs. 74.3% for P4). Later in life, when the sample sizes were smaller (and thus standard errors were greater), the pattern reversed; survival of P3 females from age 7 to age 8 was 64%, whereas survival of P4 females from age 7 to age 8 was 70%. Unlike Reiter and Le Boeuf's linear regression on grouped data, the CPHM takes into account variation in sample size that could potentially bias results. We find Reiter and Le Boeuf's results compelling but feel that a larger data set (or different data set) should be analyzed to confirm the trends they report.
Future Fecundity and Breeding Intensity
W. J. Sydeman et al. (1991) investigated the effects of prior reproductive effort on current and future reproductive success for northern elephant seal females. To index previous reproductive effort, the number of prior parturi-
tions in a female's reproductive history was tallied. The number of prior parturitions for each female is equivalent to a female's "experience." It is important to note that experience not only serves as an estimate of yearly accrual of breeding skill but also reflects physical acts of reproduction. As such, experience, as measured, is an index to potentially costly reproductive activities such as gestation and parturition. The effects of "experience" on weaning success were considered, with the potentially confounding effects of female age, using multiple logistic regression.
Weaning success increased asymptotically with increasing female age (fig. 8.1a), but this relationship was clouded by the relationship between the number of times a female had previously pupped, that is, experience, and weaning success (fig. 8.1c). By statistically adjusting for the experience effect, W. J. Sydeman et al. demonstrated that the relationship between female age and weaning success was actually log-linear (fig. 8.1b), indicating that weaning success improved with each increase in female age. The relationship between female age and weaning success indicated that the benefits of increasing age were steadily realized throughout a female's lifetime, a conclusion also reached by Reiter, Panken, and Le Boeuf (1981), although these authors did not control for the confounding effects of experience.
Weaning success also improved with increasing female experience, but this relationship appeared to plateau and decline for extremely experienced animals (fig. 8.1c). Reiter, Panken, and Le Boeuf (1981) also found that weaning success improved with increasing experience for young animals. By statistically correcting for the effect of female age (as discussed above), the downturning in the relationship between experience and weaning success became more pronounced (fig. 8.1d). Thus, the benefit of increasing experience diminished throughout an animal's lifetime, and effects became negative after five prior parturitions. The interaction between female age and female experience, that the effect of experience was dependent on age, was also significant. Another way to view this result can be seen when considering experience effects within age groups. For example, among animals aged 4 and 5, the effect of experience on weaning success was positive (regression coefficient = .714), whereas for females aged 11 through 15, the effect of experience was negative (regression coefficient = –.279).
Results suggest that when females are young, experience is beneficial, whereas late in life (after five previous reproductive bouts), additional experience is detrimental. This result is not counterintuitive if one considers that experience may be an index to costly reproductive activities such as gestation, parturition, and, in some cases, lactation. Thus, the relationship between experience and weaning success demonstrates a cost of prior reproductive effort to future fecundity. However, the negative relationship between experience and weaning success was found only after adjusting for the effect of female age; that manipulation deserves clarification. Biologi-
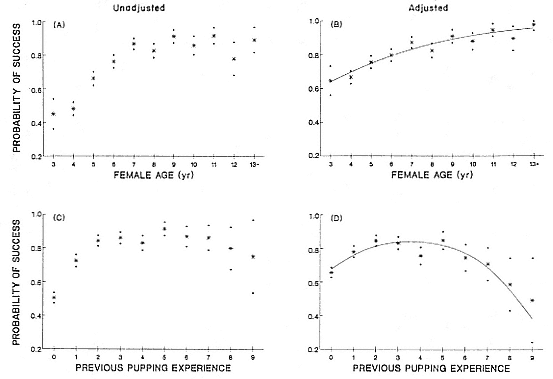
Fig. 8.1
Age-specific weaning success (A) before and (B) after adjustment for previous pupping experience.
Experience-specific weaning success (C) before and (D) after adjustment for female age.
Data are means ± 1 SE. Results of logistic regression analyses are shown (linear in B , quadratic in D ).
Reprinted, by permission, from Sydeman et al. 1991.
cally, age and experience would be the same measurement if all females began to reproduce at the same age and gave birth yearly. Due to the aforementioned variation in both age at first breeding and natality/intermittent pupping, this was not the case. Experience is actually equal to:
age — age at first breeding — the number of breeding seasons skipped .
Therefore, experience adjusted for female age reflects the effects of variation in age at first breeding and intermittent reproduction. Because experience adjusted for age reflects these life history traits, results are supportive of a trade-off in the life history strategies of female northern elephant seals; females that first breed at too young an age, or too intensely (continuously rather than intermittently), pay a cost in terms of reproductive success late in life. Studies on red deer (Clutton-Brock, Albon, and Guinness 1989) and birds (reviewed by Nur 1990) also demonstrate costs of reproduction expressed as reductions in future fecundity.
Population Dynamics and the Cost of Reproduction
Reiter and Le Boeuf (1991) investigated age-specific weaning success and optimal age at first breeding in relation to rookery density and juvenile survivorship. These data allowed them to examine whether the benefit of breeding at age 3 outweighs the cost, expressed as the decrement in survival. Age-specific weaning success was greatest in low-density rookeries, and density effects were most influential on young animals. Reiter and Le Boeuf used these data, combined with information on age-specific survivorship, to calculate the net reproductive rate and intrinsic rate of increase for populations composed of either P3 or P4 females and to estimate reproductive value of P3 versus P4 females. To model population growth, juvenile survivorship to the age of recruitment was assumed to be either 40% or 80%; results of the model depend heavily on which juvenile survivorship value is used. Model projections indicate that at either low or high density, P4 females maintain greater reproductive value at each age class than P3 females. Estimates of the intrinsic rate of increase for P3 versus P4 populations indicated higher growth rates for the P4 group.
We converted the intrinsic rate of increase to the finite (annual) growth rate (l ) using the formula l = er , where r = the intrinsic rate of increase (table 8.1). Because elephant seal populations do not grow continuously through time but grow in a discrete manner, we believe that l provides a more useful and easily interpretable metric than the intrinsic rate of increase (r). The population generated by P3 females in a high-density rookery with low (40%) juvenile survival was decreasing at a rate of 14.8% per annum compared to the P4 population, which declined at 11.4% per annum. The population generated by P3 females in a low-density rookery with 40% juvenile survival was decreasing at 8.1% per annum, compared
|
to a decline of 5.9% per annum for the P4 population. Except for conditions of low density and extremely high (80%) juvenile survival, model populations were declining. Declining model populations are consistent with field data from AN and SEFI indicating that immigration maintains current populations and that internal recruitment is very low (Le Boeuf and Reiter 1988; Huber et al. 1991). For example, Huber et al. estimate that only 17% of the females born on SEFI return there to breed.
Reiter and Le Boeuf (1991) conclude that at very low densities, for example, for an incipient colony where weaning success and juvenile survival is high, breeding at age 3 may be optimal, but under other circumstances, P4 is optimal. Northern elephant seals at AN have very low success at rearing pups as 3-year-olds, and thus it is not surprising that the benefit of breeding at age 3 did not outweigh the cost. This conclusion is consistent with field data from both colonies, where the modal age at first reproduction is 4 years. Also, if P3 is selected against, the frequency of 3-year-old breeders should be declining (if age of primiparity is heritable). Notably, on SEFI, fewer than 15% of the animals are primiparous at age 3 (Huber et al. 1991; Sydeman et al. 1991). We would expect a similar decline in P3 females at AN for recent years. Finally, Reiter and Le Boeuf's analysis points to the adaptive value of dispersal and immigration to small or newly formed colonies when high-density conditions prevail at a natal rookery.
Significance of Individual Variation for Life History Patterns
Understanding the evolution of reproductive strategies requires understanding variance , in addition to the mean , in reproductive success. Hence, an analysis of individual differences in weaning success is called for (Gillespie 1977; Seger and Brockmann 1988). Here we address the possibility of differ-
ences among females as well as differences within individuals between years. To this end, in addition to an analysis of mean weaning success, we provide an analysis of the distribution of successes and failures for each individual. Many investigators have looked at the total number of young weaned (or for birds, fledged), but no one, to our knowledge, has examined the pattern of successes and failures during an individual's lifetime.
First, as an introduction to this analysis, we consider what proportion of the variation in weaning success is attributable to female age and breeding experience and what proportion to differences among individuals. We restricted our data set to those females with a history of at least three years of reproductive success. Without controlling for age or experience, we calculated that the "individual effect" accounted for approximately 26% of the variation in weaning success (F = 1.47, df = 120, 500, p = .003, r2 = .261). Controlling for age and experience, we found that the individual effect accounted for approximately 24% of the variation in weaning success (F = 1.49, df = 120, 495, p = .0019, partial r2 = .235). Age and experience, combined, explained an additional 12% of the variation in weaning success (F = 17.3, df = 4, 493, p < .001, partial r2 = .115). This analysis indicates that some females are consistently better and others consistently worse at raising their pups to weaning age. Additionally, the effect of differences among individual females on weaning success is independent of the effects of age and experience, and, notably, between-individual differences in weaning success had greater predictive powers than age or experience in determining reproductive success.
Next, we compared the distribution of successes and failures with a null model that assumes that all individuals of a given "longevity" class have the same probability of successful reproduction. Biologically, this implies that there is no variation (i.e., homogeneity) among individuals in the probability of success in a given year. Differences among individuals (i.e., heterogeneity) would be expressed as excess numbers of individuals that are either very successful or very unsuccessful in rearing pups to weaning age. Second, the null model assumes that there is independence among the reproductive attempts within an individual. Biologically, this implies that success or failure in one attempt does not affect success or failure in a following attempt. If there is within-individual dependence in pup-rearing ability, it could be of a compensating or depensating nature. For example, T. H. Clutton-Brock et al. reported that individual red deer, Cervus elaphus , hinds that were barren or unsuccessful at rearing a calf in one year were more likely to successfully rear a calf in the following year. Interannual dependence of this nature is compensating and would lead to less variation in the distribution of successful and unsuccessful weaning among females than expected under a null model. Alternatively, Le Boeuf, Condit, and Reiter (1989) found that female northern elephant seals that failed to wean a pup
|
in one year were less likely to rear a pup in the next year. Interannual dependence of this nature is depensating and would lead to excess variation in the distribution of successful and unsuccessful weaning among females than expected under the null model. Thus two factors, heterogeneity among individuals and depensation, lead to excess variance, while only one factor, compensation, leads to a deficit of variance relative to that expected in a history of successes and failure of a given animal. Depensation is essentially a positive feedback mechanism between successive breeding attempts, while compensation represents a negative feedback mechanism.
We first revisit the question, are there differences among individuals in the likelihood of success? We use the Farallon distributional data set to answer it. We summarized successes and failures in relation to the number of breeding records per female from 1978 to 1989 in table 8.2. Each individual female appears only once in the summary. The number of records per individual is an index of longevity because it represents the number of records per individual for which we have information on successful or unsuccessful weaning, not the true reproductive life span of females. Only for longevity classes 2, 3, 4, and 5 (table 8.3) do we have adequate data to test individual females by comparing observed and expected numbers of successes and failures. For longevity class 2, we found that the variation in weaning success per individual was equal to that predicted by the null model. For longevity classes 3, 4, and 5 considered separately, we found that the observed distribution of success and failure tended to be slightly excessive
|
|
relative to expected values (0.20 > p > .10). By pooling the data for females with three, four, and five years of reproductive history, we found significant heterogeneity among individuals (c2 = 7.66, df = 3, p = .054). There were more animals that succeeded completely or that failed totally than expected by the null model (fig. 8.2).
To examine the significance of heterogeneity among individuals, compensation, or depensation, we calculated the observed and expected variance in weaning success for each longevity class assuming homogeneity among
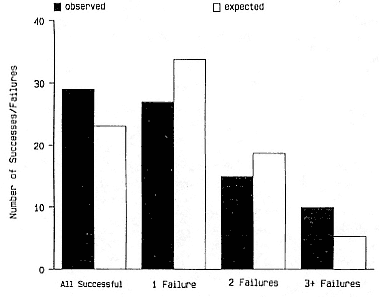
Fig. 8.2
The observed and expected number of successes and failures for
female northern elephant seals on SEFI, California, for which
we had 3 to 5 years of reproductive history. For example,
"one failure" represents females who were successful in
weaning a pup 2 out of 3, 3 out of 4,
and 4 out of 5 times.
individuals and no compensation or depensation within individuals (table 8.4). The final column indicates the direction and magnitude of the deviation between the observed and expected variance. Up to longevity class 5, the difference between observed and expected variance is positive, whereas after class 5, the difference is negative. The change in the sign indicates excess variation, relative to the null model, for females with five or fewer years of reproductive history and minimal variation for animals with six or more years of information. The negative discrepancy between expected versus observed variance for animals appearing often in the data set can only be explained by dependence of successes and failures within an individual that is of a compensating nature; that is, failure in one year promotes success in the next and vice versa (Clutton-Brock, Albon, and Guinness 1989). However, the positive discrepancy in the observed versus the expected variance for animals appearing relatively infrequently in the data set could be attributed to either heterogeneity among individuals or depensation.
One hypothesis, that excess variation among "longevity" classes 3, 4, and 5 is attributable to heterogeneity among individuals, predicts that "poor quality" individuals, that is, those most likely to fail, and those that contribute much of the excess variation in longevity classes 3, 4, and 5, will
|
drop out of the data set late in life. If so, longevity should be significantly correlated with weaning success. Indeed, weaning success increased significantly with increasing "longevity" (table 8.4; logistic regression analysis; LRSlinear = 33.63, p < .001; LRSquadratic = 3.92, p = .048); however, after controlling for age, the effect of "longevity" was no longer significant (LRS = 1.57, p = .210). The same negative result was obtained after controlling for experience (LRSlongevity = 0.74, p = .390). Therefore, the longevity effect can be attributed to age and/or experience; longevity adds little predictive power beyond the effect of age or experience in determining weaning success. Supporting this result is a comparison of the reproductive success of "short-lived" females and "long-lived" females during second and third breeding attempts only. Females destined to be long-lived were no more successful as young breeders than females destined to be short-lived (table 8.5). Together, these results provide evidence against the prediction that poor quality individuals are less likely to survive to the oldest longevity classes in our data set. Instead, these results support the concept of depensation, rather than heterogeneity among individuals, as an explanation for the excess variation observed in weaning success for animals appearing five or fewer times in the Farallon data set. For northern elephant seal females, we conclude that (1) early in life there appears to be depensating dependence between reproductive attempts (as suggested by Le Boeuf, Condit, and Reiter 1989), but later in life there is compensating dependence (as in Clutton-Brock, Albon, and Guinness 1989), and (2) heterogeneity among individuals is not related to longevity.
Conclusion
In the literature, there is considerable evidence of trade-offs in the life history of female northern elephant seals. These trade-offs involve both future fecundity and survival. In terms of future fecundity, the cost of reproduction is related to continuous rather than intermittent reproduction and reproduction at a young age versus deferred breeding (Sydeman et al. 1991). Conceptually, the cost may be detailed as follows: late in life, individuals who have bred often for their age, that is, those that show high levels of
breeding intensity, have poorer reproductive success than those who have bred fewer times for their age. We believe that age of primiparity plays a more significant role than continuous reproduction in determining future fecundity, but additional research is needed to address this issue. The exact mechanism associated with reduced weaning success for elderly females is unknown, but a decrease in aggressiveness or dominance may play a role in declining pup survival. In terms of survival, the cost of reproduction appears to be related to age at first breeding (Reiter and Le Boeuf 1991) and possibly natality (Huber 1987). Maternal survival was inversely related to age of primiparity (Reiter and Le Boeuf 1991), although we have remarked that additional data are needed to confirm statistical significance of differences in survival rates. Calculations of reproductive value and the intrinsic rate of population increase (Reiter and Le Boeuf 1991) and estimates of annual population growth rates (this chap.) indicate that deferred breeding to age 4 is optimal. Based on this model, P3 females should be decreasing in frequency in the California population, a suggestion consistent with field observations from the Farallones (Huber et al. 1991). However, due to the frequency of ENSO events in the 1980s and changes in population density, arguments concerning the match between optimal age of primiparity and field observations remain speculative.
We also investigated the potential "individual female" effect on weaning success directly in this chapter. Results indicate that some females are better and others are worse at raising their pups to weaning age. In fact, the individual female effect accounted for more of the variation in mean weaning success than maternal age and breeding experience combined. The individual female effect may be due to choice of parturition site or correlates of phenotypic variation, such as body size. Excess variation in the distribution of successes and failures among relatively short-lived females on the Farallones was probably related to depensation rather than heterogeneity among individuals, although direct between-year, within-individual comparisons are needed to address this hypothesis. The concept of depensation in northern elephant seals is supported by the findings of Le Boeuf, Condit, and Reiter that resightings of females that had successfully reared a pup were significantly greater than resightings of females that failed to rear a pup and that females that had experienced reproductive failure in one year were no more likely to be successful in the subsequent year. The deficit in variation observed for females appearing six or more times in the Farallon data set can only be explained by compensation.
The individual female effects described herein also have application to life history theory and estimates of cost of reproduction for this species. Models of alternative reproductive strategies need to be viewed in the context of differences among individuals because such variation may affect estimates of the cost of reproduction (Nur 1988; Partridge 1989). For example, if females of superior quality show higher reproductive rates than females of
inferior quality, and if reproductive effort is optimized in relation to quality, then a positive correlation between maternal survival and weaning success could arise despite there being a survival cost associated with increased reproductive effort (Nur 1988). If individual factors influence weaning success, it is likely that these same factors will influence female survival. The result of such confounding is that a survival cost associated with reproductive effort may be masked by differences in female quality. Indeed, the lack of statistical significance in survival rates between P3 and P4 females, as determined by us using the CPHM, may be explained by this type of confounding by individual variation.
Individual female northern elephant seals may be following mixed reproductive strategies (Maynard Smith 1982) governed by individual quality. Breeding late in life or intermittently may be part of a life history characterized by low reproductive effort and longevity. Conversely, early breeding and continuous reproduction may characterize a life history of high reproductive effort at the expense of longevity. Direct modeling of fitness curves for these or other alternative strategies would offer insight. With continued investigations on marked populations of northern elephant seals, the causes and consequences of variation in life history patterns in a varying environment may be better understood.
Acknowledgments
We thank the Farallon Patrol of the Point Reyes Bird Observatory for providing transportation to and from the Farallon Islands. We acknowledge and thank the staff of San Francisco Bay National Wildlife Refuge–U.S. Fish and Wildlife Service, managers of the Farallon Islands, for their help and encouragement in conducting these studies. Harriet Huber and Steve Emslie deserve special recognition for their supervision of fieldwork and data management and David Ainley, for his interest and foresight in establishing the Farallon project. Numerous field biologists participated in this study; to all, we offer our sincere appreciation. Results and interpretations of this study were sharpened by critical reviews and discussions with Burney Le Boeuf, Joanne Reiter, Jim Estes, Roger Gentry, and Gerry Kooyman. This is PRBO contribution no. 529.
References
Clutton-Brock, T., ed. 1988. Reproductive Success: Studies of Individual Variation in Contrasting Breeding Systems . Chicago: University of Chicago Press.
Clutton-Brock, T. H., S. D. Albon, and F. E. Guinness. 1989. Fitness costs of gestation and lactation in wild mammals. Nature 337: 260–262.
Gillespie, J. H. 1977. Natural selection for variances in offspring numbers: A new evolutionary principle. American Naturalist 111: 1010–1014.
Glantz, M. H., and J. D. Thompson, eds. 1981. Resource Management and Environmental Uncertainty: Lessons from Coastal Upwelling Fisheries . New York: John Wiley and Sons.
Huber, H. R. 1987. Natality and weaning success in relation to age of first reproduction in northern elephant seals. Canadian Journal of Zoology 65: 1131–1316.
Huber, H. R., A. C. Rovetta, L. A. Fry, and S. Johnston. 1991. Age-specific natality of northern elephant seals at the South Farallon Islands, California. Journal of Mammalogy 72: 525–534.
Kalbfleisch, J., and R. L. Prentice. 1980. The Statistical Analysis of Failure Time Data . New York: John Wiley and Sons.
Le Boeuf, B. J., and K. T. Briggs. 1977. The cost of living in a seal harem. Mammalia 41: 167–195.
Le Boeuf, B. J., R. Condit, and J. Reiter. 1989. Parental investment and secondary sex ratio in northern elephant seals. Behavioral Ecology and Sociobiology 25: 109–117.
Le Boeuf, B. J., and J. Reiter. 1988. Lifetime reproductive success in northern elephant seals. In Reproductive Success , ed. T. Clutton-Brock, 334–362. Chicago: University of Chicago Press.
Maynard Smith, J. 1982. Evolution and the Theory of Games . Cambridge: Cambridge University Press.
Nur, N. 1988. The cost of reproduction in birds: An examination of the evidence. Ardea 76: 155–168.
———. 1990. The cost of reproduction in birds: Evidence from manipulative and non-manipulative studies. In Population Biology of Passerine Birds: An Integrated Approach , ed. J. Blondel, A. Gosler, J. D. Lebreton, and R. McCleery, 281–296. Heidelberg: Springer Verlag.
Partridge, L. 1989. Lifetime reproductive success and life-history evolution. In Lifetime Reproductive Success in Birds , ed. I. Newton, 21–440. London: Academic Press.
Reiter, J., and B. J. Le Boeuf. 1991. Life history consequences of variation in age of primiparity in northern elephant seals. Behavioral Ecology and Sociobiology 28: 153–160.
Reiter, J., K. J. Panken, and B. J. Le Boeuf. 1981. Female competition and reproductive success in northern elephant seals. Animal Behavior 29: 670–687.
Schaffer, W. M., and M. L. Rosenzweig. 1977. Selection for optimal life histories. II. Multiple equilibria and the evolution of alternative reproductive strategies. Ecology 58: 60–72.
Seger, J., and H. J. Brockmann. 1988. What is bet-hedging? In Oxford Surveys in Evolutionary Biology , vol. 4, ed. P. H. Harvey and L. Partridge, 182–211. Oxford: Oxford University Press.
Stearns, S. C. 1976. Life-history tactics: A review of the ideas. Quarterly Review of Biology 51: 3–47.
Sydeman, W. J., H. R. Huber, S. D. Emslie, C. A. Ribic, and N. Nur. 1991. Age-specific weaning success of northern elephant seals in relation to previous breeding experience. Ecology 72: 2204–2217.
Williams, G. C. 1966. Natural selection, costs of reproduction, and a refinement of Lack's principle. American Naturalist 100: 687–690.
Nine—
Sexual Selection and Growth in Male Northern Elephant Seals
Walter L. Clinton
ABSTRACT. I studied the interactions between body size, growth, and life history in male northern elephant seals, Mirounga angustirostris , by constructing a growth curve and comparing the characteristics of growth to the pattern of male life history. The aim of this study was to determine the relationship between growth rate and age-specific male mortality rates. Four related exponential functions and a two-component logistic function were fitted to age-length data by nonlinear least squares regression. The two-component logistic curve fit the age and length data better than the best fitting exponential curve, the Richards function; however, both functions indicated a peak in growth rate around 3 to 5 years of age. Growth rates were high from 2 to 6 years of age, with relative growth rates of about 10% per year. Standard length of males increased each year of life until 9 years of age, and measurements of actual yearly growth indicated that after physical maturity, males stopped growing.
The peak in growth rate around 3 to 5 years of age and the end of growth by 9 years of age were related to important characteristics in the life history of males. The growth spurt may be associated with delayed maturity and a consequence of sexual selection for large body size. The timing of the growth spurt coincided with the lowest age-specific mortality rates over the life span of males and with the ages when increased growth rate was matched by longer periods of foraging at sea. Thus, the ages when males were exposed to the survival disadvantages of high growth rates were actually a period of low mortality. The high mortality among males occurred at 9 to 10 years of age after growth has ended and appeared to be associated with competition for mates.
Growth rates and body size are correlated with life history parameters such as age at maturity and age-specific fertility (Calder 1984). Where sexual selection has produced sexual dimorphism with males larger in size, male life history characteristics have also changed: males mature more slowly than females, delay breeding to older ages, and die at a higher age-specific
rate (Trivers 1985). To study the interactions between body size, growth, and life history, I collected data on size and age of male northern elephant seals, M. angustirostris , and constructed a growth curve to compare the characteristics of growth with those of male life history (Clinton 1990; Clinton and Le Boeuf 1993).
Modifications of pinniped male growth patterns due to sexual selection have been analyzed mainly by comparison with the growth pattern of females (Laws 1953; Scheffer and Wilke 1953; Carrick, Csordas, and Ingham 1962; Bryden 1972; Innes, Stewart, and Lavigne 1981). These studies show that males undergo an adolescent growth spurt, a type of pattern that was first described in humans (Bogin 1988; Harrison et al. 1988). In southern elephant seals, M. leonina , growth curves differ early between the sexes (Carrick, Csordas, and Ingham 1962). Males are larger than females after 1 year of age, and from 2 to 4 years of age, males grow at a faster rate than females. Growth curves indicate the female growth rate steadily decreases after 1 year of age. After a period of decreasing growth rate, the males' growth rate increased at 6 to 7 years of age, which is one to two years after puberty. This growth pattern in pinniped males was also found in northern fur seals, Callorhinus ursinus , in which the growth rate accelerated one to two years after puberty (Scheffer and Wilke 1953).
In polygynous species in which males are larger than females, the high male growth rates seen near puberty may be associated with increased male mortality rates (Ralls, Brownell, and Ballou 1980). The aim of this study was to determine the relationship between growth rate and age-specific male mortality in northern elephant seals. First, I review male life history to establish the age-specific pattern of male mortality; second, I present the growth curve for males; and finally, to consider whether increased growth rate is associated with increased mortality, I examine the concurrence between increased growth rate and changes in age-specific male mortality.
Review of Male Life History
The life history of male northern elephant seals has been strongly shaped by sexual selection (Le Boeuf and Reiter 1988; Clinton 1990; Clinton and Le Boeuf 1993). Compared to females, males delay the age of first breeding and live shorter lives. Most important, male reproductive success depends on the ability to compete for matings. Males that obtain the most matings presumably incur the greater risks and expend more energy during breeding competition (Deutsch, Haley, and Le Boeuf 1990); thus, increases in male reproductive success should be associated with decreased survival and decreased future mating success.
The life table indicates the key periods during the life of males and reveals the effects of sexual selection (table 9.1) (Clinton 1990; Clinton and
|
Le Boeuf 1993). Males reached phenotypic maturity and began to mate at ages ranging from 5 to 10 years, with a mean age of 8 years. Male fecundity increased until 12 years of age, which is very late in the short fourteen-year male life span. After an initial decrease over the first four years of life, male mortality rates increased steadily from 5 to 10 years of age, a period that included the ages when males are growing in size and developing their secondary sexual characteristics. But at 11 to 12 years of age mortality rates dropped, even though fecundity continued to increase.
The second drop in mortality rates produced a characteristic "hump" in the middle of the age-specific mortality curve. Two factors possibly contributing to this increased mortality are relatively young and inexperienced males entering reproductive competition and nutritional stress due to high male growth rates (Ralls, Brownell, and Ballou 1980). Male elephant seals fast during the breeding season, and the length of the fast increases sharply at 6 years of age (fig. 9.1). Part of the increase in male mortality may be attributed to young males increasing the length of their breeding haul-out and changing its timing so that it overlaps with the period of female estrus.
In male northern elephant seals, reproduction early in life is costly in terms of survival and contributes to the increase in mortality in males from 6 to 8 years of age. A phenotypic cost of mating is shown by the negative relationship between current mating success and future mating success at these ages (table 9.2) Males that mated at 7 to 8 years of age had poor
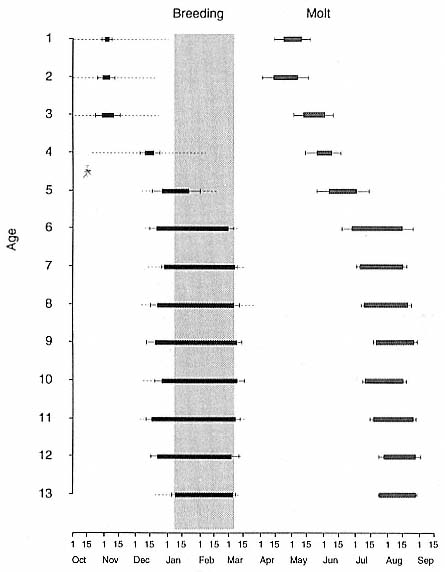
Fig. 9.1
The annual cycle of males from 1 to 13 years of age. The black and gray bars show
the dates and duration of mean haul-out periods for the breeding season and molt
season. The broad gray band indicates the period when females are in estrus.
The error bars are the standard deviation of the haul-out duration, and the dashed
lines indicate the standard deviation of the arrival and departure dates (when these
exceeded the standard deviation of the haul-out duration).
|
prospects because their mortality was higher during the year after breeding compared to males who did not mate (Clinton and Le Boeuf 1993). At 9 years of age, the relationship between current mating and future mating success diminished, then shifted to a positive but not significant relationship at the ages when mortality rates decreased. The cost of mating was evident during the ages when males were not yet phenotypically mature, and high male growth rates before maturity may have contributed further to the increased mortality rate. To determine the growth rate of males, I collected length data from a cross section of males from weaning to 14 years of age.
Methods of Measuring Length and Growth
Measuring the Length of Male Elephant Seals in the Field
From 1983 to 1988, serial nose-to-tail length measurements of 117 known-age males and 103 tagged males of unknown age were obtained at Año Nuevo, California, during the fall haul-out or winter breeding season (Clinton 1990). Tagged males were measured each season they were present whenever this was possible. Individual seals were measured more than once in a season so that measurement error could be assessed. Attempts to obtain multiple measurements focused primarily on known-age males. Altogether, 437 length measurements were obtained in the field.
Male seals were measured with a tape measure when lying at rest with a straight body posture on flat sand or gravel. Straight line measurements from nose to tail were taken as males lay on their bellies or sides. Body position (lying on belly or side) and body posture (straight or tail curved) were recorded. According to the American Society of Mammalogist's (1967) definition of standard length for seals, the males ought to have been measured
while lying on their backs. This was not possible with unrestrained seals, and males on their sides usually appeared to have the same general posture as an animal on its back, so measurements of individuals in both positions, lying on the belly and lying on the side, were obtained to measure the effect of body position on the length measurement.
Field measurements were adjusted to a set of standard conditions that were equivalent to the measurement of length that would have been obtained if a seal was on its side. Based on multiple measurements in different positions, males measured while lying on their bellies had 4.7 cm added to their length to compensate for the slight decrease in length obtained due to this body position. These adjusted standard lengths are simply referred to as standard lengths in the text.
Means and standard errors of standard length, growth rate, and relative growth rate were calculated for individual males grouped by age. Actual yearly growth of individual males was measured by subtraction of consecutive standard lengths for each seal measured in consecutive seasons, and relative growth was calculated by dividing annual growth by the initial standard length. Based on repeated measurements of individuals during the same season, I estimated the error of length measurements to be ±10.8 cm, which is 2 to 3% of a male's length (Clinton 1990); the magnitude of measurement error limited the accuracy of measured yearly growth for individual males, especially as growth rates neared zero.
To increase the sample size for males at older ages, 14 tagged adult males, 10 to 13 years old, were included in the cross-sectional data. These males were tagged as subadults and could be aged to within ±1 year based on the developmental categories that each seal was assigned as they returned to Año Nuevo each season (Le Boeuf 1974; Clinton 1990; Clinton and Le Boeuf 1993).
Exponential Growth Curves
A family of four exponential functions—the Richards, the logistic, the Gompertz, and the Brody curves (table 9.3)—were fitted to these data to estimate the parameters of the growth curve (Brody 1945; Richards 1959; Fitzhugh 1975). These functions are commonly used because their parameters have useful biological interpretations (Richards 1959). The equations are written so that length (L) is a function of age, but since these curves are based on the idea that growth rate depends on an organism's current size, growth and relative growth are functions of length. The important features of the male growth pattern, such as the changes in growth rate with age, the age of highest growth rate, and the age when growth ends, were obtained from the parameters of the function that best fitted the data. The important parameters are m, k, and A because my objectives are to describe the growth pattern of male seals to adult size (which is related to m
|
and k) and to estimate when growth ends (which is related to A). The value of m determines the shape of the curve and the size (or age) when growth rate is maximal. If m > 0 the age-length curve will be sigmoid, and the growth rate will have a maximum at some age after the beginning of the curve. The value of k determines the inhibition of growth as age increases; basically, if m, b, and A are constant, larger k values mean larger size at given ages during the period of growth, faster growth at young ages, and a faster approach to asymptotic size. Because b is a time scaling factor, its value is not biologically significant.
A sigmoidal curve describes growth in most birds and mammals. This growth pattern is usually modeled with the Richards curve, or two of the special cases of this curve—the logistic and the Gompertz curves (Richards 1959; Case 1978; O'Connor 1984; Sibly and Calow 1986). The Richards equation is a four-parameter function that encompasses the three-parameter functions, since each of the other curves can be derived by setting m to specific values: m = 2 for the logistic, and m ® 1 for the Gompertz. Where growth decreases steadily after birth, the best fit will be obtained when m = 0, which is the Brody function. Each of these functions has a different shape mainly because each has a different point of inflection, which is the length (or age) at which growth rate is highest.
Multicomponent functions based on the logistic function have been used widely in recent longitudinal studies of growth curves that featured growth
spurts (Bock et al. 1973; Koops 1986). The change in growth rate is modeled by an additional set of parameters that begin to contribute to the shape of the growth curve at the transition between stages in a multicomponent function. In this study, the cross-sectional data for male M. angustirostris were fitted to a two-component function consisting of the sum of two logistic functions:
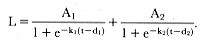
The first part of this equation governs growth early in life, and the second part begins to contribute to size later in life. The parameters d1 and d2 are the ages of the maximum growth rate for the first and second components; these parameters also eliminate the scaling parameter b from the function. For the cross-sectional data in this study, maximum growth in the first phase was assumed to occur near birth when pups are nursing from their mothers, so d1 was set to zero initially before estimating the other parameters.
The exponential growth functions were fitted to the cross-sectional age-length data by nonlinear least squares regression using the SAS NLIN procedure (SAS Institute 1985). All standard length measurements obtained for aged males were included in the data set. Strictly speaking, the data were mixed cross-sectional–longitudinal since some individuals were measured at more than one age (Fitzhugh 1975). Although data for these seals violated the least squares assumption that the observations are independent, the estimates of age-specific size and growth rate are usually improved by collecting mixed data instead of obtaining purely cross-sectional data (Tanner 1951).
Growth of Male Northern Elephant Seals
Standard length of males increased each year of life until 9 years of age. Table 9.4 shows the age distribution of males measured and the descriptive statistics for standard length, growth, and relative growth. Yearly growth rates were high from 2 to 6 years of age, and the relative growth rates indicate males increased their linear size by adding about 10% per year to their length until growth slowed at 7 years of age. But changes in growth rate in young males are not reflected in these data since 2- to 5-year-old males were difficult to measure in consecutive seasons, which limited the sample sizes for growth at each age to one to two males. The curves fitted to the length and age data had larger samples for these ages, and the growth rates of young males could be calculated from the parameters of the age-length curve.
To determine the exponential function that best described the rela-
|
tionship between age and standard length, the four related exponential curves were fitted to the cross-sectional age and length data by nonlinear least squares regression. Since the variances in standard length of males at each age were not equal (Bartlett's test: B = 23.951, df = 13, p < .05), the data for each age were weighted by the inverse of the standard deviation of length at that age. The Richards function with an m value close to 3 was the best fitting function of the exponential family of curves (table 9.5). The next best exponential function was the logistic curve, where m = 2, and 3 parameters are estimated by least squares rather than 4 parameters. A likelihood ratio test (Gallant 1987) of the Richards function versus the logistic function indicated the Richards function was a better fit of the data (L = 3.393, P(L) = P[F1,171 ] = 0.933, P is the likelihood that the full model is a better fit).
The two-component logistic curve fitted the age and length relationship of the cross-sectional data better than the Richards function according to several criteria. The two-component logistic curve had a higher R-square value (table 9.5). Although a reliable F test evaluating the significance of the difference between the fit of these two curves is not available, a pseudo-F test (variance ratio) indicates an improved fit of the two-component logistic curve versus the Richards function (F1,171 = 18.233). The plot of cross-sectional data with the two-component curve showed that this curve closely
|
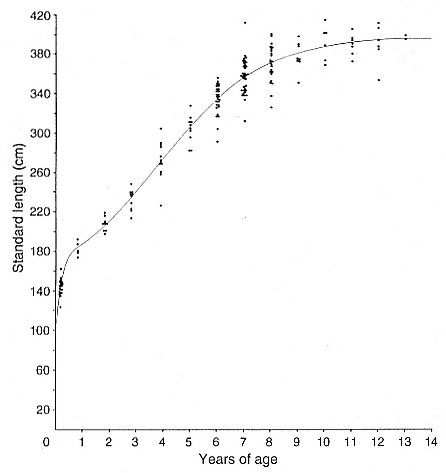
Fig. 9.2
The two-component logistic growth curve plotted with the
cross-sectional data for male age and standard length.
followed the data (fig. 9.2). In comparison with the yearly growth data that were collected, the two-component logistic function was closer to measured growth rates among young males, and both functions were good predictors of growth after 5 years of age. Since the growth rate predictions of the functions differed most at these younger ages, the better fit of the two-component logistic curve indicates that it was a better model of male growth during the ages of high growth rates.
At 9 years of age, both exponential functions for the age and length curves were within 95% of asymptotic size, and the instantaneous yearly growth rate was about

to approach the zero growth rate, but male seals appear to stop growing by 9 years of age (table 9.4). Measurements of yearly growth indicated that 7 years of age was the last year of significant measurable growth (H0 : µ = 0,



Life History and Growth
The Richards and the two-component logistic growth curves both showed two important features of the growth pattern for male northern elephant seals, which were the peak in growth rate around 3 to 5 years of age and the end of growth after 8 years of age. These two characteristics in the growth pattern relate to the life history of males in this species. First, increased growth rates of males near puberty have been found in other polygynous seals and in anthropoid primates in which males are larger than females in size, males mature and reproduce later in life than females, and the mating systems are polygynous. This suggests that particular modifications in the growth patterns, such as a growth spurt near puberty, may be associated with sexual selection for large body size in males and that the acceleration in growth rate around puberty may be connected to delayed maturity. Second, growth in length ends when maturity is reached at 8 to 10 years of age and males make the transition to the ages of successful social competition for mates (Clinton 1990; Clinton and Le Boeuf 1993).
High growth rates around puberty were first noted in domesticated animals and in humans by S. Brody (1945). He suggested that the postnatal growth rate increased until puberty and then decreased, producing a sigmoidal growth curve such as the Richards curve. Except for the primate growth models with an adolescent growth spurt, the commonly used growth models have been simple exponential functions with a sigmoidal shape; primate growth models have required multicomponent functions to account for the more complicated pattern of growth featured by some primates (van Wagenen and Catchpole 1956; Bock et al. 1973; Froehlich, Thorington, and Otis 1981; Glassman et al. 1984). In some pinniped species (Laws 1953;
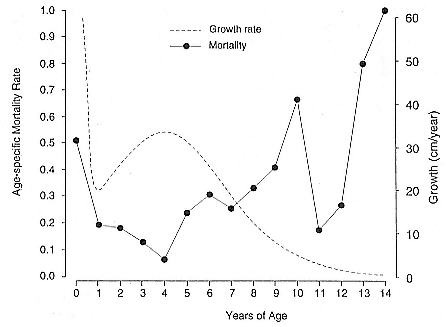
Fig. 9.3
The male growth curve versus the age-specific male mortality curve (modified
from Clinton and Le Boeuf 1993), showing that there is no direct relationship
between growth rate and mortality rate.
Scheffer and Wilke 1953; Carrick, Csordas, and Ingham 1962; Bryden 1972), the male growth pattern also features a growth spurt, and in this study of M. angustirostris , a two-component logistic growth function yielded the best model of male growth. In a recent reanalysis of growth studies in pikas, mice, and rabbits, W. J. Koops (1986) found that the multicomponent logistic functions provided a "nearly perfect" fit to longitudinal growth data that had originally been modeled with simple exponential functions. This raises the question, if growth patterns in mammals are better modeled with more complex functions, then why have simple exponential functions provided realistic and reliable models for so many studies? Koops suggests that part of the reason is the difficulty in collecting the data needed to distinguish the number of growth phases. Also, the simple exponential functions have fitted growth data well enough to model growth in species other than those with large, obvious growth spurts. In pinnipeds, where large size is strongly favored, selection for high growth rates in males appears to have accentuated the differences between growth phases by increasing the rates at the peak periods of growth during later stages of growth.
At 4 years of age, the timing of the growth spurt in northern elephant
seals coincided with the age when age-specific mortality rates were at their lowest over the life span of males (fig. 9.3). The high growth rate during the growth spurt does not appear to affect survival of males. Instead, the relationship between the life history of males and growth rates indicates that high growth rates occur when the energetic costs of growth may be at their lowest. Growth rates are very high during nursing, when the pup is provided milk with a very high energy content by the mother (Ortiz, Costa, and Le Boeuf 1978). The high growth rates from 3 to 5 years of age coincide with the period in the annual cycle of males that has short periods of fasting onshore during the fall and, conversely, longer periods of foraging at sea. By the ages when males begin to fast for long periods during the breeding season, the growth rate has diminished. Thus, increased growth rates coincided with periods when the increased energetic costs of higher growth rates were more likely to be matched by increased feeding rates. This result is surprising, since higher growth rates have been reasonably assumed to be a costly disadvantage of large male size, which is thought to be favored by sexual selection and opposed by increased mortality. Such an effect is not likely in northern elephant seals. The period when males ought to be most exposed to the survival disadvantages of high growth rates is in fact a period of low mortality, and the level of natural selection is at a low point during this period. Instead, the highest mortality among males occurs at the ages after growth has ended. Selection mostly takes place on males that have attained full size. Thus, the size attained during growth would be the character potentially affected by selection rather than the growth rate.
References
American Society of Mammalogists. 1967. Standard measurements of seals. Journal of Mammalogy 48: 459–462.
Bock, R. D., H. Wainer, A. Petersen, D. Thissen, J. Murray, and A. Roche. 1973. A parameterization for individual human growth curves. Human Biology 45: 63–80.
Bogin, B. 1988. Patterns of Human Growth . Cambridge: Cambridge University Press.
Brody, S. 1945. Bioenergetics and Growth . New York: Hafner Publishing Company. Reprinted 1968.
Bryden, M. M. 1972. Growth and development of marine mammals. In Functional Anatomy of Marine Mammals , ed. R. J. Harrison, 1–79. London: Academic Press.
Calder, W. A., III. 1984. Size, Function, and Life-History . Cambridge: Harvard University Press.
Carrick, R., S. E. Csordas, and S. E. Ingham. 1962. Studies on the southern elephant seal, Mirounga leonina (L.). IV. Breeding and development. CSIRO Wildlife Research 7: 161–197.
Case, T. J. 1978. On the evolution and adaptive significance of postnatal growth rates in the terrestrial vertebrates. Quarterly Review of Biology 53: 243–282.
Clinton, W. L. 1990. Sexual selection and life history of male northern elephant seals. Ph.D. dissertation, University of California, Santa Cruz.
Clinton, W. L., and B. J. Le Boeuf. 1993. Sexual selection's effects on male life history and the pattern of male mortality. Ecology 74: 1884–1892.
Deutsch, C. J., M. P. Haley, and B. J. Le Boeuf. 1990. Reproductive effort of male northern elephant seals: Estimates from mass loss. Canadian Journal of Zoology 68: 2580–2593.
Fitzhugh, Jr., H. A. 1975. Analysis of growth curves and strategies for altering their shape. Journal of Animal Science 42: 1036–1051.
Froehlich, J. W., R. W. Thorington, Jr., and J. S. Otis. 1981. The demography of howler monkeys (Alouatta palliata ) on Barro Colorado Island, Panama. International Journal of Primatology 2: 207–236.
Gallant, A. R. 1987. Nonlinear Statistical Models . New York: John Wiley and Sons.
Glassman, D. M., A. M. Coelho, Jr., K. D. Carey, and C. A. Bramblett. 1984. Weight growth in savannah baboons: A longitudinal study from birth to adulthood. Growth 48: 425–433.
Harrison, G. A., J. M. Tanner, D. R. Pilbeam, and P. T. Baker. 1988. Human Biology 3d ed. Oxford: Oxford University Press.
Innes, S., R. E. A. Stewart, and D. M. Lavigne. 1981. Growth in Northwest Atlantic harp seals, Phoca groelandica. Journal of Zoology, London 194: 11–24.
Koops, W. J. 1986. Multiphasic growth curve analysis. Growth 50: 169–177.
Laws, R. M. 1953. The elephant seal (Mirounga leonina , Linn.). I. Growth and age. Falkland Islands Dependencies Survey, Scientific Reports 8: 1–62.
Le Boeuf, B. J. 1974. Male-male competition and reproductive success in elephant seals. American Zoologist 14: 163–174.
Le Boeuf, B. J., and J. Reiter. 1988. Lifetime reproductive success in northern elephant seals. In Reproductive Success , ed. T. H. Clutton-Brock, 344–362. Chicago: University of Chicago Press.
O'Connor, R. J. 1984. The Growth and Development of Birds . Chichester: John Wiley and Sons.
Ortiz, C. L., D. Costa, and B. J. Le Boeuf. 1978. Water and energy flux in elephant seal pups fasting under natural conditions. Physiological Zoology 51: 166–178.
Ralls, K., R. L. Brownell, and J. Ballou. 1980. Differential mortality by sex and age in mammals, with specific reference to the sperm whale. Report of the International Whaling Commission Special Issue 2: 233–243.
Richards, F. J. 1959. A flexible growth function for empirical use. Journal of Experimental Botany 10: 290–300.
SAS Institute. 1985. SAS User's Guide: Statistics . Ver. 5. Cary, N.C.: SAS Institute.
Scheffer, V. B., and F. Wilke. 1953. Relative growth in the northern fur seal. Growth 17: 129–145.
Sibly, R. M., and P. Calow. 1986. Physiological Ecology of Animals . Oxford: Blackwell Scientific Publications.
Tanner, J. M. 1951. Some notes on the reporting of growth data. Human Biology 23: 93.
Trivers, R. L. 1985. Social Evolution . Menlo Park, Calif.: Benjamin/Cummings Publishing Company.
van Wagenen, G., and H. R. Catchpole. 1956. Physical growth of the rhesus monkey (Macaca mulatta). American Journal of Physical Anthropology 14: 245–273.
Ten—
Sex- and Age-related Variation in Reproductive Effort of Northern Elephant Seals
Charles J. Deutsch, Daniel E. Crocker, Daniel P. Costa, and Burney J. Le Boeuf
ABSTRACT. The aim of this study was to determine how reproductive effort (RE) varies with age and sex in the northern elephant seal, Mirounga angustirostris . RE is an important feature of life history that links the proximate costs with the fitness costs of reproduction. Percentage of mass lost over the breeding season provided an energetic index of RE because both sexes fast on the rookery. We summarize research conducted over an 11-year period at Año Nuevo, California. Seventy-three females ranging in age from 3 to 12 years were chemically immobilized and weighed during the lactation period, providing 22 measurements of maternal mass loss. Males ranging in age from 5 to 13 years were weighed (N = 56), or their mass was estimated using a photogrammetric technique (N = 94), yielding 87 measurements of breeding mass loss.
The principal findings were as follows. 1 . The magnitude of RE was similar between males and females. Adults of both sexes lost slightly more than one-third of their mass, on average, despite large sex differences in reproductive strategy and body size. Adult males were an average of 1.4 times longer and 3 to 4 times heavier (mean ± SD = 1,814 ± 233 kg, range = 1,430–2,550 kg) than adult females (488 ± 80 kg, range = 360–710 kg) at the start of the breeding season. Male mating effort was more variable than female parental effort, and some bulls lost up to half of their arrival mass. The timing and intensity of RE differed between the sexes: males fasted three times longer than females, but they incurred less than one-third the mass-specific mass loss rate of females. Males suffered vastly more external injuries from conspecifics than did females, yet females were probably at greater risk of receiving life-threatening internal injuries. 2 . Female effort during lactation was not correlated with maternal age or mass, but gestation effort (i.e., relative neonatal mass) declined with increasing maternal mass. Absolute measures of investment in offspring—including maternal mass loss, neonatal mass, weanling mass, and pup mass gain—were directly proportional to maternal mass, which increased from 3 to 6 years of age before reaching an asymptote. Maternal investment in sons was similar to that in daughters. 3 . Males delayed serious efforts at mating until age 6, two to three years later than females but still physically
immature, and mean RE was subsequently constant with age. Dominant males devoted a greater proportion of body stores to breeding and obtained higher mating success than did subordinates.
The similarity in RE of growing "subadults" and physically mature breeders for both sexes was contrary to theoretical expectations, considering the poorer reproductive success and higher fitness costs of the former. Possible explanations include the existence of a threshold RE below which fitness costs are minimal, the higher fitness benefit of early-born offspring in an expanding population, and the benefit of experience to future reproductive performance.
One can, in effect, treat the sexes as if they were different species, the opposite sex being a resource relevant to producing maximum surviving offspring.
—R. L. Trivers (1972)
Males and females reproduce in fundamentally different ways, and the resulting divergent selective pressures have led to numerous sex differences in morphology, physiology, life history, and behavior (Trivers 1972, 1985; Glucksman 1974; Clutton-Brock, Guinness, and Albon 1982). A crucial aspect of mammalian life history that has rarely been compared quantitatively across the sexes is reproductive effort (RE). Typically defined as the proportion of available time and energy that an organism allocates to reproduction over a specified period of time (Gadgil and Bossert 1970; Hirshfield and Tinkle 1975; Tuomi, Hakala, and Haukioja 1983), RE also includes a component of risk that is important but more difficult to quantify (Calow 1979; Warner 1980). Reproductive effort can be divided into two categories: mating effort, which involves expenditure of time, energy, or risk to obtain matings (e.g., searching for mates, fighting for dominance or territory necessary for access to mates); and parental effort, which involves such expenditure to produce, nurture, and raise offspring (Low 1978). Herein lies a major difference between the RE of males and of females. In the majority of mammals and in most polygynous species, males exhibit little or no parental care, so that virtually all male RE is mating effort (Alexander and Borgia 1979). In contrast, females invest considerably in their young, especially in mammals, which exhibit extended postnatal care and nurture offspring via the energetically expensive process of lactation (see Loudon and Racey 1987). Consequently, the selective pressures that have shaped the pattern of RE should differ between the sexes. Female RE is thought to have evolved primarily in response to ecological and demographic factors, whereas the evolved pattern of male RE has apparently been strongly influenced by the mating system and the intensity of male-male competition for mates (Trivers 1972; Warner 1980; Thornhill 1981).
The principal reason for the paucity of empirical studies contrasting male and female RE in polygynous vertebrates is that male mating effort has been neglected (Stearns 1976; Warner 1980; Gittleman and Thompson
1988). Furthermore, sex differences in energy intake during the breeding season and in risks associated with reproducing (e.g., Ryan 1985) often make it difficult to measure RE in a comparable way for each sex (Knapton 1984). Ideally, one would study a species in which breeding is temporally separated from foraging and other activities related to growth and survival. Then essentially all activity would serve a reproductive function, all energy expended above maintenance levels would be devoted to reproduction, and energy intake would be nil or absent. Such conditions are found in some phocids, such as elephant seals and gray seals, in which both sexes fast and remain on land during the breeding season, relying on stored energy reserves to fuel their metabolic and reproductive needs. Studies on phocid reproductive energetics were initially conducted on females and pups due to their smaller size, greater site predictability, and relevance of the work to parental investment theory (Fedak and Anderson 1982; Ortiz, Le Boeuf, and Costa 1984; Stewart and Lavigne 1984; Costa et al. 1986; Stewart 1986; Bowen, Boness, and Oftedal 1987; Hill 1987; Tedman and Green 1987; McCann, Fedak, and Harwood 1989; Kovacs, Lavigne, and Innes 1991; Hammill et al. 1991; Bowen, Oftedal, and Boness 1992; Fedak et al., this volume; see review by Oftedal, Boness, and Tedman 1987). Logistic difficulties involving the capture and weighing of large, aggressive, and mobile animals have hindered research on male reproductive energetics in pinnipeds until recently (Anderson and Fedak 1985; Reilly 1989; Deutsch, Haley, and Le Boeuf 1990; Boyd and Duck 1991; Reilly and Fedak 1991; Twiss 1991; Bartsh, Johnston, and Siniff 1992; Fedak et al., this volume; Walker and Bowen, in press).
We studied sex- and age-related variation in reproductive effort of the highly polygynous and sexually dimorphic northern elephant seal, M. angustirostris , in central California. Sex differences in life history and behavior are numerous in this species (see Le Boeuf 1981; Le Boeuf and Reiter 1988; Le Boeuf and Laws, this volume). Age at primiparity ranges from 2 to 6 years, with most females initially giving birth at age 3 (36%) or age 4 (54%) at Año Nuevo (Reiter and Le Boeuf 1991). Females produce one pup annually thereafter, with occasional skipping (Huber 1987), until death at a maximum age of 19 years. Male growth is extended by two to three years, and reproduction is delayed compared to females. Males attain sexual maturity by age 5 and rarely skip breeding seasons subsequently (Clinton 1990); yet due to intrasexual competition, most do not mate until physical maturity at age 8 or 9 (Clinton, this volume), five years later than the age of first mating for most females. The maximum life span recorded for a male is 14 years.
Considerable interest in optimal life history theory has focused on identifying the age-specific pattern of reproduction that maximizes fitness for
a given set of demographic conditions and constraints (see Charlesworth 1980). Assuming that reproduction imposes a cost, measured as a reduction in future fitness (Bell 1980; Bell and Koufopanou 1985), there should be a level of RE at each age that optimizes trade-offs between current and future reproduction (Williams 1966a , 1966b ; Gadgil and Bossert 1970; Pianka and Parker 1975). A frequently asserted prediction is that RE should increase with age as residual reproductive value declines; that is, since older individuals generally have less to lose in terms of future offspring than younger ones, investment should be high late in life (Williams 1966b ; Gadgil and Bossert 1970; Pianka 1976; Caswell 1982). This trend is favored by low population growth rate, low extrinsic adult mortality rate, and continuous increase in body size or reproductive efficiency during adulthood (Charlesworth 1980). The opposite conditions theoretically favor a decline in RE with age. In rapidly expanding populations, for example, breeding should occur as soon as physiologically possible (Lewontin 1965; Cody 1971; Stearns 1976), and young adults should exhibit the greatest effort (Charlesworth and León 1976). Though studies on this point among long-lived birds and mammals are few, there is some empirical support for increasing (Clutton-Brock 1984; Pugesek 1981, 1983, 1984; Hamer and Furness 1991; Pärt, Gustafsson, and Moreno 1992; but see Nur 1984), decreasing (Stewart 1986), and constant (Reid 1988) RE with age; other studies have yielded mixed results (Green 1990).
The expected ontogenetic pattern of resource allocation to reproduction must be considered separately for each sex. Reproductive value of female northern elephant seals declines gradually from primiparity to age 11 and then drops steeply to the end of the life span (Reiter and Le Boeuf 1991), suggesting that the fitness cost of reproduction should also decrease with age, especially among the oldest animals. Weaning success increases with age and experience, particularly from sexual maturity to physical maturity at age 6 (Reiter, Panken, and Le Boeuf 1981; Reiter and Le Boeuf 1991; Sydeman et al. 1991). This combination of rising benefits and declining potential costs of breeding with age suggests that maternal effort should increase with age. However, the current high population growth rate (Cooper and Stewart 1983; Stewart et al., this volume) enhances the fitness value of early-born offspring relative to those born later in life, and this will favor high effort early in life. Given these opposing forces, it is difficult to predict how female RE should change with age, although we expect a high terminal investment when residual reproductive value is low (i.e., > 10 years old). Since natality rate is high and relatively constant with age after the initiation of reproduction (Le Boeuf and Reiter 1988; Huber et al. 1991), our study focused on the RE of parous females and did not consider the frequency of barren years.
For male elephant seals, sexual selection is likely to have shaped the agespecific pattern of RE. Intense intrasexual competition among males in highly polygynous species should favor a lifetime mating strategy involving little investment when young and a high mating effort after attaining a reproductively competitive size (Warner 1980; Maher and Byers 1987). Male elephant seals have a relatively short effective breeding life span (age 8–13), with mating success rising precipitously on reaching physical maturity (Le Boeuf and Reiter 1988; Clinton and Le Boeuf 1993). Since subadult males are growing, any allocation of time and energy to reproduction might reduce their growth rate (e.g., Green and Rothstein 1991), final adult size (e.g., Boyce 1981), and, hence, future fecundity. Therefore, we predicted that male mating effort should be low during the subadult period (age 4–7) when the fitness benefit of reproductive investment is nil and potential costs are high and that effort would increase abruptly at adulthood when bulls are able to fight effectively for high dominance rank and access to mates. Contrary to expectation, C. J. Deutsch, M. P. Haley, and B. J. Le Boeuf (1990) found that older subadults (6–7 years old) expended the same RE (i.e., percent mass loss) as low-ranking adult males.
In this chapter, we compare reproductive effort between male and female northern elephant seals as indicated by mass loss while breeding (energy component), duration of stay on the rookery (time component), and incidence of external injuries (risk component). Our objectives are to: (1) summarize existing data on body mass as a function of age for both sexes, much of which is currently unpublished or scattered across a number of publications; (2) compare the magnitude of male mating effort with female parental effort, in terms of relative mass loss, time commitment, and apparent risk; and (3) describe age-specific patterns of RE for both sexes and relate them to changes in reproductive success and mortality with age.
Methods
General Methods and Study Area
The studies summarized here were conducted from 1981 to 1991 at Año Nuevo State Reserve, 70 km south of San Francisco, California. Most work was done on the expanding mainland colony (see Le Boeuf and Kaza 1981 for a description of the area), which increased over the study period from 300 to 1,500 breeding females distributed among 8 to 16 harems. The annual maximum in number of sexually mature males present on the Año Nuevo mainland during the breeding season (December to March) fluctuated between 300 and 500, of which about one-third were adults. Individuals were identified over the course of the breeding season by applying cream bleach or black hair dye to the pelage. Numbered plastic tags placed
in the webbing of the hind flippers provided information on age and permitted identification of individuals from year to year.
Exact ages were unknown for most males in the study. Males were assigned to one of five age classes based on their overall size and on the development of secondary sexual characters (neck shield and proboscis), both of which correspond with age to within about one year (Le Boeuf 1974; Cox 1983; Clinton 1990): subadult-one (SA1) males = 4 years old; subadult-two (SA2) = 5 years; subadult-three (SA3) = 6 years; subadult-four (SA4) = 7 years; and adult males (AD) = 8–14 years. Most observations concern the last three age classes, since there were few SA1 and SA2 males present during the breeding season. Known-age adult males and those with known histories were further classified as either young adult (first- or second-year adult, approximately 8–9 years of age) or old adult (at least third-year adult, 10 years and older).
Copulations and dominance interactions among males were recorded in the field using standard methods, and the estimated number of females inseminated provided a measure of male mating success (Le Boeuf 1974). Dominance ranks of adult males were determined in each year using the Bradley-Terry method (Boyd and Silk 1983; Haley, Deutsch, and Le Boeuf, in press), and these values were used to assign males to one of three rank classes.
Measurement of Body Mass and Mass Loss
Females
One or more weights were obtained on 73 females and their pups near the beginning and/or the end of the female's lactation period. Sixty-one mothers were of known age, ranging from 3 to 12 years old. The sample includes female-pup pairs weighed during the course of studies on diving behavior (Le Boeuf et al. 1986, 1988, 1989) and reproductive energetics (Costa et al. 1986; Crocker 1992; D. Costa and B. Le Boeuf, unpubl. data). An additional 18 pups were weighed within three days of birth and again within six days of weaning, 17 of whose mothers were of known age (Kretzmann 1990; Kretzmann, Costa, and Le Boeuf 1993). The 91 motherpup pairs included 9 females that were experimental subjects in two or more years, giving a total of 79 different females. After chemically immobilizing a lactating female, she and her pup were weighed with a tripod scale (accurate to ± 2.5 kg) and standard length measurements were taken (Costa et al. 1986). For the energetic studies, 22 different female-pup pairs were weighed 0 to 5 days postpartum, and the procedure was repeated an average of 21.2 ± 2.0 days later (range = 18–25 days), within a few days of weaning. Methods are given in more detail in D. P. Costa et al. (1986) and D. E. Crocker (1992). Data on energy expenditure, body composition, and milk intake are presented elsewhere (Ortiz, Le Boeuf, and Costa 1984; Costa et al. 1986; Crocker 1992; Kretzmann, Costa, and Le Boeuf 1993).
Females that abandoned or lost their pup after experimental manipulation (N = 5), or who regularly nursed more than one pup (N = 2), were excluded from calculations of maternal mass loss, departure mass, lactation duration, pup mass gain, and pup mass and length at weaning.
Males
Body mass was obtained or estimated for 140 different males (n = 247 measurements of mass) ranging in age from 5 to 13 years and in dominance status from low-ranking to alpha male. Estimates of daily and seasonal mass loss are presented for 82 males (n = 87 measurements) and 71 males (n = 75 measurements), respectively. The data on male body mass come from two studies.
1. In 1988 and 1989, 56 males were weighed on a platform scale (accurate to + 0.25%), yielding 95 weights and 30 measures of breeding mass loss over a mean ± SD interval of 32.7 ± 18.7 days (range = 9–83 days). One male was anesthetized; the rest were either lured onto the scale using a model of a female elephant seal and playback of female vocalizations or moved onto the scale using tarpaulins and playback of male threat vocalizations. Details of weighing methods and results appear in Deutsch, Haley, and Le Boeuf (1990).
2. Body mass was estimated for an additional 94 males at the start and/or the end of the 1989 breeding season using a photogrammetric technique that predicts mass with a 95% confidence interval of ± 14% (r2 = .93; Haley, Deutsch, and Le Boeuf 1991). This provided 152 estimates of mass and 57 measures of mass loss over a mean interval of 58.0 ± 13.3 days (range = 42–96 days) (Deutsch 1990).
Since there were no significant differences in daily or seasonal mass loss (absolute and mass-specific) of males between the direct weighing and photogrammetric methods (t-tests for each age class, p > .05), the two data sets were pooled for presentation here. Photogrammetric estimates of adult male body mass at arrival and departure were significantly greater, however, than were the extrapolated figures based on direct measurements (arrival: 1,851 ± 236 vs. 1,710 ± 200 kg, t = 2.09, df = 61, p < .05; departure: 1,227 ± 144 vs. 1,098 ± 115 kg, t = 3.72, df = 75, p < .001). This probably reflects the fact that large, high-ranking males (which were difficult to weigh) comprised a greater proportion of the photographic data set than the direct weight data set. The two methods of measuring mass did not differ significantly for SA3 males (p > .05), but photogrammetric estimates were significantly greater than direct estimates for SA4 males (arrival: 1,539 ± 234 vs. 1,274 ± 140 kg, t = 2.26, df = 11, p < .05; departure: 1,106 ± 158 vs. 870 ± 107 kg, t = 3.81, df = 18, p < .01). This mass difference was apparently not due to a methodological discrepancy, because the
photographic sample of SA4 males was indeed larger in standard length than the weighed sample (p < .05). Standard length was measured photogrammetrically in both studies (Haley, Deutsch, and Le Boeuf 1991).
Incidence of Injuries
Injuries provide one index of risk taken in reproduction because they represent the degree to which the animal was exposed to potentially damaging or lethal interactions while reproducing (Deutsch 1990; Le Boeuf and Mesnick 1991). Wounds can get infected and, if severe, can reduce fecundity (e.g., Clutton-Brock et al. 1979; Le Boeuf, Riedman, and Keyes 1982) or lead to death (e.g., Laws 1953; Wilkinson and Shank 1976). The incidence of external injuries was measured by recording the number, location, and severity of fresh wounds on males (1989) and females (1991) during the main mating period in February. Wounds were considered fresh if they were open, bleeding, or oozing and thus represented recent injuries. This analysis was limited to injuries inflicted by conspecifics and excluded shark-inflicted wounds.
Data Analysis
Values of body mass are presented at arrival (or parturition for females) and departure dates to standardize comparisons across ages and sexes. Only direct measurements of mass and breeding mass loss are used in the sex comparison. Descriptive statistics are presented as mean ± one standard deviation (SD). For nine females and nine males that were sampled in two or more years, mean values of size, mass change, and other variables were calculated for each individual; these values were then used in the calculation of overall means by sex or age class (e.g., table 10.3). For correlations between RE variables and size or age, however, each measurement was treated as an independent data point (e.g., table 10.4). In these analyses, P-values were determined after reducing the degrees of freedom to equal the number of individuals (rather than measurements) in the sample minus the number of estimated parameters (see Sydeman et al. 1991). The level of statistical significance was set at a = .05 for all tests.
Measures of the effort devoted to reproduction (e.g., mass loss, pup mass gain) are presented here in absolute and mass-specific terms as a function of age and sex. RE should be expressed as a fraction of the organism's ability to invest (e.g., proportion of energy stores or body mass). However, since proportions can sometimes be misleading when the dependent variable varies allometrically with body mass (Packard and Boardman 1987), we adjusted for the potentially confounding effect of body mass on indexes of RE using one of three additional methods: (1) analysis of covariance (ANCOVA) for comparison of male mass loss by age class and for comparison of maternal investment by sex of pup; (2) partial correlations between
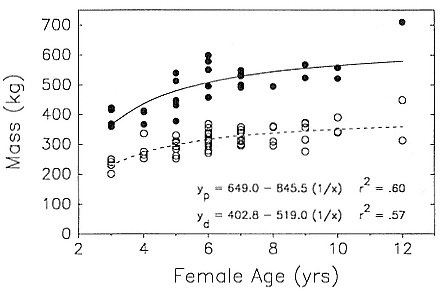
Fig. 10.1
Breeding female mass at parturition (solid circles, N = 28) and at departure from the
rookery (i.e., weaning) (open circles, N = 54) as a function of maternal age. Both
regressions are significant (p < .0001). The heavier departure mass at age 12
is a rough estimate (see footnote c in table 10.3).
maternal investment variables and age, controlling for female mass; and (3) correlation of residuals (from the regression of maternal investment indexes against parturition mass) with maternal age. Plots of residuals were inspected visually for homoscedasticity (i.e., constant variance of residuals around the regression line), and the normality of residuals was checked with the univariate procedure in SAS (SAS Institute 1985). An arcsinesquare-root transformation was applied to percentages before performing parametric statistical tests (Sokal and Rohlf 1981). Descriptions of how mass, mass loss, and other variables were calculated are presented in appendix 10.1.
Results
Sexual Size Dimorphism
The most striking difference between the sexes in elephant seals is their size. At arrival, adult males weighed an average of 3.5 (range = 2–7) times more than adult females (table 10.1; see figs. 10.1 and 10.2). Estimates of arrival mass for adult males varied from 1,430 kg to 2,265 kg for weighed animals and up to 2,550 kg for a photographed animal. Departure mass of
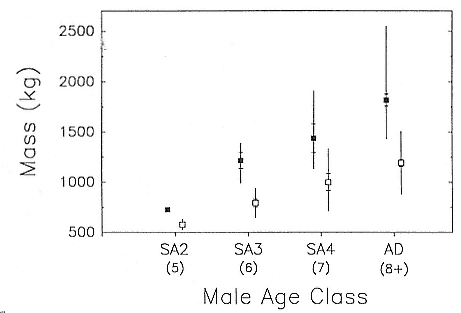
Fig. 10.2
Male mass at arrival (solid squares) and at departure (open squares) from the
breeding rookery as a function of age class. Approximate age in years of each class is
shown in parentheses. Symbols show the mean (squares), 95% confidence interval
of the mean (hatch marks), and range; a 95% confidence interval is not shown
for the SA2 class due to the small sample size. Sample sizes for arrival and
departure mass, respectively, are as follows: SA2 (N = 1,2);
SA3 (N = 12, 18); SA4 (N = 11, 18); Adult (N = 62, 75).
adult males ranged from 895 to 1,500 kg (fig. 10.2). Female mass at parturition (i.e., immediately postpartum) ranged from 360 to 710 kg, and departure mass ranged from 200 kg to at least 400 kg (fig. 10.1; table 10.3). Given that males and females arrive at different times and subsequently lose mass until departure, the mass ratio between an adult male and a lactating female at any given time could range from about 1.5 to 10. However, a three- to sixfold dimorphism in mass would be most common for a typical copulating pair in early February.
Standard length (SL) gives a more straightforward measure of sexual size dimorphism because it does not fluctuate over the annual cycle as does mass. Mean SL of 89 physically mature males measured photogrammetrically in this study was 382 ± 15 cm (range = 350–420 cm), which is similar to the mean of 385 ± 18 cm found by W. L. Clinton (this volume). Physically mature females (i.e., 6+ years of age) measured in this study had a
mean SL of 265 ± 8 cm (range = 248–282 cm, N = 32 individuals). Thus, mature males were an average of 1.44 times longer (range = 1.24–1.69) than mature females.
Both sexes became reproductively active while still growing. Females giving birth for the first time at age 3 had attained approximately 75% of mature mass (fig. 10.1, table 10.3), whereas 6-year-old males (SA3 class) were roughly two-thirds the mass of mature bulls (fig. 10.2, table 10.5). The cross-sectional data on size and a limited amount of longitudinal data indicate that females stopped growing in length and mass at age 6 (parturition mass = 541 ± 58 kg, N = 16, ages 6–12 years) and males reached a physically mature size at 8 to 9 years, growing little or not at all in length thereafter (Clinton, this volume).
Sex Comparison of Reproductive Effort
A comparison of the energetic component (breeding mass loss) and the time component (duration of the breeding fast) of RE for adult male and female elephant seals is presented in table 10.1. The most important finding was that the mean proportion of mass lost over the breeding season was very similar for both sexes (36–37%), suggesting that the energetic component of RE while ashore was also similar. A proximate reason for this outcome was that the greater intensity of the females' parental effort (i.e., percent body mass lost per day) compared to the males' mating effort was balanced by a longer duration of stay for males. Duration of the breeding fast for adult males was about three times longer than that of females (table 10.1). Relative mass loss over the season was significantly more variable for adult males (range for weighed animals = 26–46%) than for females (range = 31–41%) (variance-ratio test, F = 5.78, df = 12, 21, p<.01).
Males clearly incurred a much greater risk of external injury than females. Nearly 90% of males surveyed in February possessed fresh wounds, compared to less than 20% of females (table 10.2). The mean number of fresh wounds per animal was 20 times greater for males than females (table 10.2); those females with open wounds usually had only one to three small cuts. Although most injuries appeared superficial for both sexes, the potential for serious injury was reflected in the percentage of seals with fresh wounds on the head (including face, nose, and eye), which again was significantly higher for males than females.
Female Reproductive Effort in Relation to Maternal Age, Mass, and Pup Sex
Energetic measures of the two components of maternal investment—gestation and lactation—are plotted as a function of maternal age, mass, and pup sex in figure 10.3 and summarized as a function of maternal age in table 10.3.
|
|
Effect of Pup Sex on Maternal Investment
Theoretical predictions (Maynard Smith 1980) and some empirical studies (e.g., Anderson and Fedak 1987; Oftedal, Iverson, and Boness 1987) indicate that females invest more in sons than in daughters in polygynous mammals. Therefore, we initially examined the data, using two-tailed t-tests, for differences in the mother's size, age, and investment or the pup's size and growth rate as a function of pup sex. Of all variables in table 10.3, only percent daily maternal mass loss was significantly different between mothers with sons (1.53 ± 0.10%, N = 10) and mothers with daughters (1.43 ± 0.10%, N = 12; t = 2.43, df = 20, p = .025). Absolute mass loss per day did not vary significantly with sex of the pup (t = 0.71, df = 20, p = .48); after adjusting for the effect of maternal parturition mass, however, mothers of male pups showed a higher mass loss rate than mothers of female pups (ANCOVA: F = 5.34, df = 1, 19, p = .032). This finding is not consistent with our other results because none of the other t-tests revealed a significant effect of pup sex on maternal investment (e.g., total mass loss, pup growth rate); analyses of covariance on the nine other absolute measures of maternal investment in table 10.3, with parturition mass as the covariate, confirmed these results (p > .10 for all tests). Furthermore, recent studies have shown a lack of differential investment in sons versus daughters in both northern and southern elephant seals (McCann, Fedak, and Harwood 1989; Le Boeuf, Condit, and Reiter 1989; Campagna et al. 1992; Kretzmann, Costa, and Le Boeuf 1993; Fedak et al., this volume). Our interpretation is that this one significant result was probably due to chance, since one comparison is expected to be significant at the p = .05 level when over 20 tests are performed simultaneously. None of the slopes defining the linear regressions of maternal investment variables on maternal mass varied significantly with pup sex (general linear models, p > .25), but the correlation coefficients were usually lower for sons than for daughters, as reflected in the greater scatter of points among small mothers of sons (see fig. 10.3e, g, i). T. S. McCann, M. A. Fedak, and J. Harwood (1989) made a similar observation for the southern elephant seal. We con-
|
|
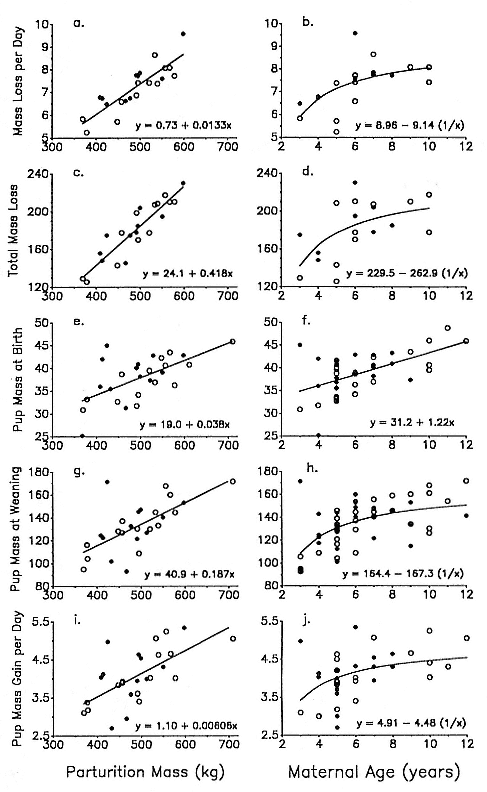
Fig. 10.3
Absolute measures of maternal investment as a function of female parturition mass and
age for male (closed circles) and female (open circles) pups. ( A, B ) Average daily mass loss
of mother. (C, D ) Total mass loss of mother over lactation period. ( E, F ) Pup mass at
birth. (G, H ) Pup mass at weaning. (I, J ) Average daily mass gain of pup. All units of
mass are in kg. See table 10.4 for sample sizes, correlation coefficients,
and levels of significance.
clude that the results do not support the hypothesis that maternal effort varies as a function of pup sex. Therefore, further analyses use the pooled data set.
Gestation Effort:
Pup Mass at Birth
Pup birth mass increased significantly with maternal mass and age (fig. 10.3e, f; table 10.4). Maternal investment during the period of gestation, as indicated by pup birth mass expressed as a fraction of mother's parturition mass, declined significantly with increasing maternal mass (y = 11.44 – 0.0075x) and maternal age (y = 9.36 – 0.234x) (tables 10.3 and 10.4). A similar negative relationship between relative neonatal mass and maternal mass is also found in interspecific comparisons among phocids (Kovacs and Lavigne 1986) and in other mammals (Millar 1977; Robbins and Robbins 1979; Gittleman 1986). Due to their smaller size, young mothers (3–5 years) put forth a greater gestation effort (pup birth mass was 8.64 ± 1.28% of maternal mass, N = 8) than physically mature, older mothers (7.43 ± 0.63%, N = 16; Wilcoxen two-sample test, z = 2.358, p < .05). Maternal age had no significant effect on pup birth mass, however, after accounting for the effect of maternal body mass (table 10.4). Analyses of pup mass at initial treatment (0–5 days postpartum) yielded similar results, although the relationship with age appeared more asymptotic.
Lactation Effort:
Maternal Mass Loss
Daily mass loss of lactating females was strongly and positively correlated with parturition mass and, to a weaker degree, with female age (fig. 10.3a, b; table 10.4). This reflects both increased absolute maintenance costs and increased mass transfer to the pup with larger maternal size (maternal mass loss per day vs. pup mass gain per day: r = .73, N = 22, p < .0001). Independent of maternal mass, age was not significantly correlated with rate of mass loss during lactation (table 10.4).
Total mass loss over the lactation period increased with female mass and age in a similar fashion (fig. 10.3c, d; table 10.4). Total percentage of body mass lost over lactation, our principal index of female reproductive effort, varied between 31 and 41% and showed no significant correlation with maternal mass or age (table 10.4, fig. 10.4). Partial correlation and residual analyses yielded the same results: age had no discernible effect on breeding mass loss after controlling for the effects of maternal mass (table 10.4).
|
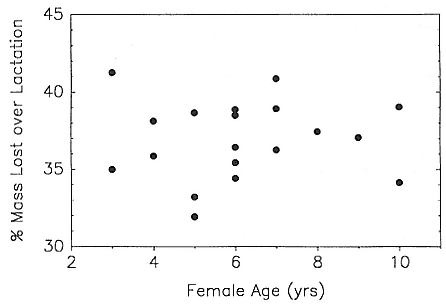
Fig. 10.4
Percentage of female parturition mass lost over the lactation period
as a function of maternal age (r = .04, N = 19, p = .87).
Lactation Effort:
Pup Mass Gain
Mass gain of pups provided another energetic index of maternal investment (e.g., Ortiz, Le Boeuf, and Costa 1984). Pup mass gain per day and over the entire lactation period rose significantly with female mass and age (fig. 10.3i, j; table 10.4). Once again, maternal age had no apparent effect on mass gain of pups after adjusting for maternal mass (table 10.4).
On average, pups gained 57.5% of the mass lost by their mothers (table 10.3). We expected that the efficiency of mass transfer would increase with maternal size since a female's mass-specific energy expenditure for maintenance metabolism (i.e., her "metabolic overhead," Fedak and Anderson 1982) should decline with increasing mass. Thus, a larger mother should be able to allocate more energy to milk production and relatively less to her own maintenance costs. The data did not support this prediction; in fact, there was a nonsignificant tendency in the opposite direction, with younger mothers being somewhat more efficient (tables 10.3, 10.4).
Lactation Duration
Duration of the lactation period averaged 25 to 26 days and was positively correlated with maternal mass (table 10.4). Mean lactation duration increased from age 3 to 5 and then remained approximately constant among older females (table 10.3). Chemical immobilization of the female near the end of lactation sometimes triggered a premature
departure, and so these figures may be biased slightly low (1–2 days at most). The relationship between duration of stay and age was generally similar to that found by J. Reiter, K. J. Panken, and B. J. Le Boeuf (1981). A comparison of experimental and control groups matched for maternal age, year of study, and breeding site showed no significant effects of manipulation on lactation duration or weanling mass (D. Crocker and B. Le Boeuf, unpubl. data).
There was an inverse relationship between the duration and intensity of female RE. Mothers that lost mass at a high daily rate, relative to their body size, nursed their pups for a significantly shorter period compared to those with a less intense maternal effort (partial r = –0.50, N = 22, p < .025, controlling for parturition mass).
Total Maternal Investment:
Weanling Mass
A pup's mass at weaning indicates the mother's total absolute investment in the young, the sum of birth mass plus mass gain during the nursing period. Weaned pup mass increased significantly with maternal mass (fig. 10.3g) and with maternal age up to 6 years (fig. 10.3h, table 10.4). At weaning, pups weighed a mean of 27.2% of mother's parturition mass (range = 20–31%, plus one outlier of 40%), and this was not significantly associated with maternal age (table 10.4). Likewise, controlling for the effect of maternal body mass on weanling mass with partial correlation and residual analyses demonstrated no effect of age independent of maternal mass (table 10.4). The outlier mentioned above may reflect milk stealing by the pup, a behavior that confounds measurements of maternal investment that rely on pup energy gain (see appendix 10.2).
Summary: Effects of Maternal Mass, Age, and Pup Sex on Female RE . Larger mothers invested absolutely but not relatively more in offspring during lactation than smaller mothers. Female RE during gestation, as reflected in relative neonatal mass, was negatively correlated with maternal age and mass. Changes in absolute measures of maternal investment with age generally tracked age-specific changes in body mass, increasing from age 3 to 6 and then reaching a plateau. Age had no discernible effect on any index of female RE (including gestation and lactation components) when the effect of maternal mass was controlled statistically. With the exception of female mass loss rate, all other indexes of maternal investment did not vary significantly with pup sex.
Male Reproductive Effort in Relation to Age, Mass, and Dominance Rank
For all ages combined (N = 71 individuals), male elephant seals lost a mean of 0.37 ± 0.08% of their arrival mass per day and 33.1 ± 7.0% of their
mass over the three-month breeding fast. Daily and seasonal mass loss increased significantly with body mass at arrival (rs = .78, N = 75 measurements, p < .0001; rs = .82, N = 75, p < .0001, respectively), with standard length (rs = .65, N = 87, p < .0001; rs = .61, N = 75, p < .0001, respectively), and with age class (table 10.5), as expected. On a mass-specific basis, however, breeding mass loss was weakly correlated with body mass (daily: rs = .21, N = 75, p = .08; seasonal: rs = .31, N = 75, p < .01) and was not correlated wlth length (daily: rs = .04, N = 75, p = .71; seasonal: rs = .05, N = 75, p = .71) or age (table 10.5). The lack of an age effect for SA3 and older classes was confirmed with an ANCOVA that showed no significant age-related variation in daily mass loss (F = 1.335, df = 2, 67, p = .27) or seasonal mass loss (F = 1.694, df = 2, 67, p = .19) when controlling for the effect of arrival mass; similar results were obtained using length as the covariate. Note that the above positive correlations between measures of mass loss and arrival mass are probably inflated due to the autocorrelation of measurement errors (i.e., an overestimate of arrival mass would cause an overestimate of both absolute and relative mass loss). Length was measured with greater accuracy than mass and showed no correlation with daily or seasonal RE (i.e., percent mass loss).
The mean duration of stay at the Año Nuevo rookery was 91.0 ± 10.9 days (range = 51–109, N = 71, excluding the SA2 male) and did not vary significantly with age among the SA3 to adult classes (table 10.5). This value may be somewhat high because our sample was probably biased against seals that departed early and were therefore less likely to be weighed or photographed a second time. There is no reason to believe that such a bias would vary with age class; Clinton (this volume) showed that average breeding haul-out duration remains fairly constant from age 6 onward.
There is a dramatic increase in male mating effort from 4 to 6 years of age, as demonstrated by the following behavioral measures: increasing duration of stay on the rookery; shifting of the haul-out period so that it overlaps the estrous period; and increasing time spent in proximity to harems (Cox 1983; Clinton 1990; Deutsch 1990; see fig. 9.1, chap. 9). Only one pubescent male (SA2 class) was weighed in this study, and he lost 27% of his mass over a 60-day period (table 10.5). Multiplying the average haul-out duration for the SA2 class (Clinton 1990: a minimum mean of 27 ± 20 days, N = 53; Deutsch 1990: mean of 56 ± 12 days, N = 6) by the mass-specific rate of mass loss for the weighed SA2 male (table 10.5) yields an estimate of 12 to 25% of arrival mass lost over the breeding fast for this age group. This suggests that young subadults devote a smaller proportion of their body stores to reproductive activity, compared to older males, by fasting for a shorter period on the rookery.
|
The highest levels of RE that we measured were exhibited by some adult males that lost nearly half of their body mass over the breeding season (maximums of 46.3% for a weighed animal and 50.5% for a photographed animal). This was considerably greater than the highest RE shown by younger males (SA3: 38.5%, weight; 35.1%, photograph; SA4: 38.8%, weight; 35.0%, photograph), perhaps because adults had the opportunity to achieve much higher mating success than subadults. The difference in sample size between the age groups provides an alternative explanation that cannot be completely ruled out, but it is unlikely that subadults ever lose much over 40% of their mass.
What can account for the twofold variation in RE among adult males? Total percent mass loss over the breeding season was slightly but not significantly higher for old adult males than for young adults (table 10.6). Age is positively correlated with dominance rank and mating success in males (Le Boeuf 1974; Le Boeuf and Reiter 1988; Clinton and Le Boeuf 1993), and the tendency for older bulls to invest more in reproduction, can be attributed to their higher rank. Although sample sizes were small, table 10.6 shows that the RE of young and old adults was quite similar for a given rank or mating success class. Males in the top third of the dominance hierarchy lost a significantly greater proportion of their arrival mass over the season (36.0 ± 7.8%, N = 23) than did bulls in the middle third (32.2 ± 5.8%, N = 18) and the bottom third of the hierarchy (32.9 ± 6.9%, N = 9) (p < .05, table 10.6). Likewise, males that achieved high mating success (ENFI > 15) devoted a greater effort to reproduction (36.3 ± 6.1% of mass lost, N = 14) compared to adults with moderate sexual success (ENFI of 5–15; 32.6 ± 9.1%, N = 13) or to those who copulated relatively few times (ENFI < 5; 33.8 ± 6.5%, N = 24). The difference in percent mass loss between males with high mating success (median ENFI = 28.8) and those with low to moderate success (median ENFI = 2.0) was not significant, however (p = .098, table 10.6).
Summary:
Effects of Age Class and Dominance Status on Male RE
Error inherent in the photogrammetric measurement of body mass created considerable variation in mass loss variables among individuals, which made it more difficult to detect the real effects of potential factors such as age or dominance status on male mating effort. Nevertheless, the following patterns stood out. 1. The energetic component of male RE increased from age 5 (SA2) to age 6 (SA3), due to increased time spent on the rookery, and then remained constant to adulthood; preliminary data indicate no effect of age on RE of adults. 2. Sexually successful, high-ranking males lost a greater proportion of body mass than did subordinate adults and subadults, but the difference in RE was small compared to the discrepancy in mating success.
|
Discussion
Comparison of Reproductive Effort between the Sexes
This study reveals important similarities and differences in the magnitude and timing of reproductive effort between male and female northern elephant seals. The most striking similarity is in the energetic index of RE, adults of both sexes losing slightly more than one-third of their body mass, on average, while on the breeding rookery. This finding suggests that a common physiological mechanism, perhaps related to changing body composition or increasing protein catabolism (Groscolas 1986; Cherel, Robin, and Le Maho 1988), may underlie the termination of the breeding fast in both sexes.
There is no a priori reason why male and female RE should be equivalent, since the factors affecting reproductive success differ so widely between the sexes in elephant seals. In males, mating success achieved for a given effort depends on the number, competitive ability, and RE of other males on the rookery (Trivers 1972; Warner 1980). Intense male intrasexual com-
petition for mates has been a strong selective force favoring continued growth and delayed maturity, thereby molding the level and developmental timing of mating effort. Consequently, young male elephant seals invest little in reproduction and much in increased growth during the first two or three years that same-age females are pupping, a common phenomenon in polygynous species (Selander 1972; Clutton-Brock, Guinness, and Albon 1982). Compared to males, competitive social interactions exert less influence on female RS (see Reiter, Panken, and Le Boeuf 1981) and, therefore, on the magnitude and ontogeny of maternal effort. Natural selection favors an investment pattern in females that optimizes the opposing effects of energy transfer to the pup on maternal and offspring fitness, relationships that are affected by fasting physiology, foraging ecology, thermoregulatory requirements at sea, and other ecological factors.
Data on mass change for four other phocid species allow comparison of RE between the sexes. The mean percentage of body mass lost over lactation is in the range of 30 to 40% for females of all five species (table 10.7). Breeding mass loss was similar for the two sexes in both species of elephant seals but higher for females than males in gray, Weddell, and harbor seals (table 10.7). The energetic cost of reproduction in the sexually monomorphic harp seal, Phoca groenlandica , appears to be much greater for females than males based on changes in blubber thickness over the breeding season (Sergeant 1973). These data suggest that only in the most polygynous seal species does male mating effort approach the level of female parental effort in terms of energetic costs.
Energy allocation to reproduction (i.e., breeding activity, gonads, gametes, and young) is far greater in female golden-mantled ground squirrels, Spermophilus saturatus , than in males (Kenagy 1987; Kenagy, Sharbaugh, and Nagy 1989), and this is probably the case for most mammals. But is this the best measure of RE? Our use of relative mass loss as an index of RE in fasting pinnipeds included energy devoted to maintenance metabolism while breeding, and so it was not a measure of reproductive energy expenditure per se. Nevertheless, since elephant seals and some other pinniped species must fast in order to breed (see Riedman 1990), it is reasonable to include this maintenance metabolic expenditure as part of the total energy cost of reproduction. Because the mating strategy of males in polygynous species often entails reduced food intake during a period of high activity, males may deplete their energy reserves to a greater extent during the mating period than females do during pregnancy and lactation (e.g., Leader-Williams and Ricketts 1981; Michener and Locklear 1990), even though actual reproductive energy expenditure may be much higher in females. Thus, we consider percent mass loss (an index of percent energy loss) while breeding to be a more appropriate measure of RE in fasting seals than energy allocation to strictly reproductive functions.
|
Differences in the pattern of reproductive effort between male and female elephant seals are related to their divergent reproductive strategies, as follows:
1. Rate of investment in reproduction, measured as mass-specific daily mass loss, was over three times higher for females than males. This has the effect of reducing the metabolic overhead costs of females relative to males. About 40% of the energy lost by females during lactation is utilized for maintenance and activity metabolism, the rest being transferred to the pup as milk (Costa et al. 1986). On the con-
trary, most of the energy males expend during the breeding season is probably allocated to maintenance metabolism since 85 to 95% of their time is spent resting (Sandegren 1976; Deutsch 1990).
2. Duration of RE was three times longer for males than females, reflecting the importance of early male arrival to obtaining high rank and of a lengthy stay to maximize chances for copulation. A consequence of this longer effort is that males had two fewer months than females to recover lost energy stores and to gain additional mass before the next breeding season. Of course, females invest energy in gestation for eight months of the year, which is not reflected in these comparisons (see Deutsch, Haley, and Le Boeuf 1990).
3. Variation in RE was greater among males than females. Male mating effort was quite flexible, as evidenced by the more than twofold variation in duration of the breeding fast and by great variability in measures of the intensity of effort, such as the proportion of time spent competing near harems (Deutsch 1990) and mass-specific rate of mass loss (Deutsch, Haley, and Le Boeuf 1990). Consequently, some young subordinate males invested considerably less than any female did, and the RE of some high-ranking males soared well above the maximum for females. In contrast, three observations indicate that flexibility in adjusting maternal effort during lactation is limited by energetic considerations. First, mothers that invested at a higher relative rate weaned their pups sooner, suggesting the existence of a cost ceiling. Second, females that lose their pups and therefore invest relatively little energy in lactation remain on the rookery longer than those who rear pups successfully (Reiter, Panken, and Le Boeuf 1981). Third, a 3-year-old mother ("Bat") who nursed two pups simultaneously lost mass at a rate similar to other females of her size (observed = 6.35 kg/day; expected = 6.25 kg/day), and the pups gained mass at a combined rate similar to single pups (observed = 3.46 kg/day; expected = 3.62 kg/day). The minimum RE of parous females would have been lower than 30% mass loss if we had included those that lost their pups and did not nurse. But since 90 to 95% of females pupping on the Año Nuevo mainland are successful in raising their pups to normal weaning age (B. Le Boeuf, unpubl. data), including unsuccessful mothers in our sample would not have altered the above finding.
This sex difference in the variance in RE mirrors the great difference in the variance in reproductive success between the sexes (Le Boeuf and Reiter 1988). Females must invest some minimum RE to produce a healthy offspring, whereas males that invest even less effort have some chance of siring a pup. Extremely high levels of maternal effort (over 40% of body mass
lost) probably benefit a pup relatively little compared to the future costs incurred by the mother, but alpha males can raise their current RS substantially through continued high mating effort. Thus, the sex difference in RE variation reflects the fundamentally different means by which male and female elephant seals maximize their RS.
Reproduction can be a risky activity, sometimes even leading to death (e.g., Wilkinson and Shank 1976; Brunton 1986), so a complete sex comparison must also examine this facet of RE. Frequency of external wounds, the measure of risk used in this study, was many times greater in males than females, reflecting the violent competition among males for high rank and access to females. Similar sex differences in frequency of injury have been found in other polygynous mammals (e.g., Smith 1966; Michener and Locklear 1990). We note, however, that the observed injury rate for females may be an underestimate because they were sampled in harems, but they are most likely to get injured during departure from the rookery. The risk of receiving a lethal injury during agonistic or sexual interactions is relatively small for both sexes at Año Nuevo; female mortality due to male-inflicted injuries (0.1% per year; Le Boeuf and Mesnick 1991) appears to be about twice as high as that for males (Deutsch 1990; C. Deutsch and B. Le Boeuf, unpubl. data). Female elephant seals probably incur a higher incidence of fatal injuries than males because of the large sex difference in mass, the large canines of males, the bites to the female's neck during typical sexual behavior, and the emaciated condition of the female on departure, when she is exposed to mating attempts by aggressive and sexually motivated peripheral bulls (Mesnick and Le Boeuf 1991).
Differences in life history parameters between the sexes may be due to differences in the timing and magnitude of RE. In Richardson's ground squirrels, S. richardsonii , for example, males deplete their fat stores to a greater degree during the mating period than females do during lactation, and this is associated with higher male mortality (Michener and Locklear 1990). The authors conclude that the costs of mating effort for males are greater than the costs of parental effort for females in this species. This pattern is taken to the extreme by the marsupial shrew, Antechinus stuartii , in which all males die soon after the mating season, whereas females are iteroparous (Lee, Wooley, and Braithwaite 1982). In northern elephant seals, the survivorship curves are roughly similar for both sexes (see fig. 22.2 in Le Boeuf and Reiter 1988), although longevity is somewhat greater for females. This is consistent with the similar magnitude of RE for the sexes found in this study.
Age-specific Reproductive Effort and Fitness Consequences
Variation in the energetic component of RE was not associated with age for males 6 years and older or for parous females. This finding, based on mass
loss, is corroborated by complementary data showing no correlation between mass-specific energy expenditure and milk energy output of lactating females and age (Crocker 1992; D. Crocker, unpubl. data) and no age-related variation in male time-activity budgets (Deutsch 1990). Unfortunately, very old females, with the lowest reproductive value and the highest predicted RE, were not included in our sample, but a follow-up study (D. Crocker, unpubl. data) found that three 11- to 12-year-old mothers lost a similar proportion of their body mass over lactation (range = 31–34%) as younger mothers. W. J. Sydeman et al. (1991) suggested that age-specific increases in female aggressiveness, success in dominance interactions, and weaning success could be attributed to increasing maternal effort with age. The energetic data do not support this interpretation; instead we propose that the increase in size and experience with age accounts for the higher dominance status and weaning success of older mothers. Even though the proportion of resources allocated to reproduction did not vary with age, it is still possible that younger, smaller seals incurred a greater risk by depleting their fat reserves to a lower, perhaps marginal, level than larger animals, as R. E. A. Stewart (1986) found for female harp seals. This was apparently not the case in elephant seals, however, since females weaned their pups on reaching 21 to 25% body fat, regardless of maternal age, parturition mass, or initial body composition (Crocker 1992; D. Costa and B. Le Boeuf, unpubl. data). Female investment in offspring was in direct proportion to maternal mass at parturition. This is similar to the pattern of maternal investment in southern elephant seals (McCann, Fedak, and Harwood 1989; Fedak et al., this volume) but unlike harp seals, in which small, young mothers produce weanlings of the same size and lose mass at the same rate as larger, older mothers (Stewart 1986; Kovacs, Lavigne, and Innes 1991).
The fitness costs of reproduction (e.g., reduced survival) should be positively related to the proximate energetic costs (i.e., RE), though probably in a nonlinear fashion, and this relationship should be manifested in the age-specific patterns of RE and mortality. This expectation is partially supported by the fact that age-specific mortality rates rise sharply upon the initiation of reproduction—at primiparity for females (age 3–4; Reiter and Le Boeuf 1991) and at puberty for males (age 5; Clinton, this volume). For females, this survival cost is associated with lactation, since mortality remains low during the year of their first pregnancy. Similarly, Clutton-Brock, Albon, and Guinness (1989) concluded that the fitness costs of reproduction in red deer, Cervus elaphus , are attributable to the energetic demands of lactation and not to gestation.
Two lines of evidence led us to expect the RE of reproductively active but physically immature "subadults" (i.e., 3- to 5-year-old females and 5- to 7-year-old males) to be less than that of physically mature animals. First, RS increases with age in both sexes, especially from puberty to physical
maturity, due largely to the effects of intrasexual competition (see references given on p. 173). Young small females obtain a lower benefit (in terms of weaning success and weanling mass) for the same proximate cost (relative mass loss) as older, larger mothers. Even more striking is the fact that subadult males obtain little immediate benefit for the same mass-specific cost as low-ranking adult males; only 6 to 15% of 6- to 7-year-olds copulate (Clinton 1990). Second, a given RE seems to impose a greater strain on the younger breeders of both sexes, manifested as higher fitness costs. Females giving birth at age 3 suffer reduced survivorship and future fecundity relative to those delaying reproduction to age 4 or 5 (Huber 1987; Reiter and Le Boeuf 1991; but see Sydeman and Nur, this volume). Current mating success is negatively correlated with subsequent survival and fecundity for young males (6–8 years old) but not for older males (Clinton and Le Boeuf 1993; Clinton, this volume). Also, C. R. Cox (1983) found that 6-year-old males that spend a greater amount of time on the breeding beach suffer higher mortality than those exhibiting lower effort. The higher fitness costs incurred by young breeders may result from the simultaneous allocation of energy to growth and reproduction, leaving less available for storage or critical maintenance functions.
Given that benefits of reproductive investment increase and fitness costs decrease with age during the period between sexual and physical maturity, selection should favor a relatively low level of RE for "subadult" animals. This probably explains why most females delay primiparity beyond age 3 (Huber 1987; Reiter and Le Boeuf 1991) and why pubescent males (4–5 years old) invest little time or energy in mating attempts (Clinton 1990; Deutsch 1990), despite the theoretical benefits of early breeding (Sibly and Calow 1986). Still, the pattern of constant mean RE with age for parous females and for males older than 5 years is perplexing. Theoretically, the optimal level of investment at each age is determined by the age-specific functions relating RE to fitness benefits (i.e., current RS) and to fitness costs (i.e., reduction in residual reproductive value). These functions are extremely difficult to determine empirically, and few data are available (Charlesworth 1980). It is noteworthy, however, that a constant age-specific RE is theoretically plausible, at least among adults, as stated by B. Charlesworth (ibid. 246): "It is not difficult, in principle, to find cases in which an optimal life-history exists which has constant reproductive effort among the adult age-classes, and hence constant adult survival and fecundity."
One could reasonably argue that a small, young female must expend a relatively large proportion of her energy stores during lactation to produce a weanling that is large enough to survive well (see Fedak et al., this volume). The point at which survival from weaning to age 2 appears to drop off is at a weaning mass of only 80 to 90 kg (Le Boeuf, Morris, and
Reiter, this volume). A hypothetical young mother of 400 kg would produce a 34-kg newborn (from fig. 10.3) and nurse it for 23 to 26 days with a mass transfer efficiency of 63% (from table 10.3). This female would lose 105 kg, or only 26% of her parturition mass, to produce a 100-kg weanling. Clearly, young mothers invest substantially more (36% of body mass) than is required to produce a viable pup. So this argument can probably be rejected; but the future fecundity of offspring may be enhanced by maternal investment above what is necessary for offspring survival. We offer three other nonexclusive explanations that may account for the lack of age-related variation in RE.
One explanation is that the seals are able to tolerate moderate energy deficiencies up to some threshold level, and only when that threshold is exceeded does a cost of reproduction become apparent (Tuomi, Hakala, and Haukioja 1983). Large fluctuations in body mass (e.g., loss of one-third mass) are a normal part of the elephant seal's annual cycle (Costa et al. 1986; McCann, Fedak, and Harwood 1989; Deutsch, Haley, and Le Boeuf 1990; Kretzmann, Costa, and Le Boeuf 1993; Worthy et al. 1992) and may entail little or no fitness costs in healthy animals. While costs associated with additional increments of investment above a critical level are likely to escalate (Burley 1988), the benefits should approach an asymptote, particularly for females. In fact, the survival benefit of large weanling mass is equivocal (Le Boeuf, Morris, and Reiter, this volume). Crocker (1992) postulated a physiological mechanism, based on changing body composition and rate of protein catabolism, that may account for a threshold relationship between maternal investment and fitness costs. Circumstantial evidence for such a threshold phenomenon includes the fact that female body fat at the end of lactation lies in a narrow range, despite the large variation in initial fat stores, in lactation duration, in absolute rates of mass loss, and in rates of lean and adipose tissue loss (Crocker 1992; see also Gales and Burton 1987). An animal exceeding the hypothesized threshold level of RE might be faced with the choice of either depleting its blubber layer beyond what is necessary for thermoregulation at sea or oxidizing muscle and other lean tissue. While this cost-based explanation is plausible, the trade-off between reproduction and growth (e.g., Robinson and Doyle 1985; Green and Rothstein 1991) would seem difficult to avoid, and so attempting to breed as a growing subadult could reduce size and, hence, fecundity as an adult (Clinton 1990; Haley, Deutsch, and Le Boeuf, in press). Furthermore, the evidence of a trade-off between reproduction and survival for young animals also argues strongly for the existence of substantial benefits, either immediate or delayed, to early reproductive attempts.
The second possibility draws on the idea that in expanding populations the fitness benefit of early-born offspring is greater than that of late-born young, thus favoring early maturity and declining RE with age (Lewontin
1965; Charlesworth and León 1976). This may partially explain why females mature at a relatively young age in M. angustirostris and in exploited populations of M. leonina (Carrick et al. 1962), although the costs of early reproduction would appear to outweigh the benefits under the prevailing demographic conditions (Huber 1987; Reiter and Le Boeuf 1991). The hypothesized effect seems too small, however, to compensate for the extremely low mating success of subadult males, unless we have grossly underestimated their success. Who mates with virgin females and where this occurs is poorly known (Huber et al. 1991) and could potentially affect our interpretation. Overall, we think that this can be a partial explanation at best.
The final explanation for the unexpectedly high RE among physically immature seals is that experience gained when young improves future reproductive performance (Caro 1988; Robinson 1988). The benefits of early maternal experience to subsequent weaning success have been documented in elephant seals (Reiter, Panken, and Le Boeuf 1981; Sydeman et al. 1991). C. J. Deutsch (1990) presents circumstantial evidence for the importance of fighting and sexual experience to males; this includes the observation that subadult males engage in frequent mock-fighting activity in contexts in which no immediate reproductive benefit is possible (e.g., during the molt period). Even male weanlings exhibit behaviors during social interactions that resemble the fighting behavior of adult males (Rasa 1971; Reiter, Stinson, and Le Boeuf 1978), suggesting that early social experience plays an important role in reproducing successfully later in life (Fagen 1981). This raises the interesting question of whether such social play among juvenile males should be considered a form of early RE with delayed benefits that has evolved via sexual selection on adult males.
In conclusion, we found that the magnitude of reproductive effort in northern elephant seals, as estimated by relative mass loss, was similar for males and females but that the temporal patterning of effort differed between the sexes both within a breeding season and over development. The similarity in RE between the sexes was unexpected, given the large size dimorphism, divergent reproductive strategies, and the generally held view that female mammals invest more in reproduction than males. RE did not vary with age in males (older than 5 years) or parous females, despite an increase in reproductive success and a decline in the fitness costs of breeding with age for both sexes. Future studies should investigate whether the substantial interindividual variation in RE is related to the amount of energy stores available at the start of breeding, thus potentially linking foraging success at sea with reproductive success on land.
Acknowledgments
Numerous students and colleagues assisted in fieldwork over the decade covered by this study, and to them we are very grateful. We also thank
M. Haley and J. Williams for their collaboration in the mass loss studies; K. Richer for collecting data on male injuries; M. Kretzmann for contributing data on pup mass and mass change; and the rangers at Año Nuevo State Park for their cooperation in the field. We are grateful to S. Blackwell, M. Bryden, R. Gentry, G. Kooyman, and J. Reiter for making helpful comments on earlier versions of this manuscript. The research was funded in part by the National Science Foundation and by the Biology Board of Studies and the Institute of Marine Sciences at the University of California, Santa Cruz.
Appendix 10.1
Calculation of Body Mass and Mass Loss
Body Mass at Arrival (Males) or Parturition (Females)
Calculated by using measured rates of mass loss for each animal to extrapolate from date of first weighing (initial mass) back to arrival date (males) or parturition date (females). For 14 males weighed (directly or photogrammetrically) only at the start of the breeding fast, initial mass was extrapolated to arrival date using the regression between mass loss per day (y) and length (where x = 0.9* standard length): y = –86.87 + 91.42x – 31.85x2 + 3.84x3 (r2 = .68, N = 28, p < .0001; Deutsch, Haley, and Le Boeuf 1990). For 12 females weighed only at the start of lactation, initial mass was extrapolated to parturition date using the regression between mass loss per day (y) and initial mass (x): y = 1.058 + 0.01306x (r2 = .62, N = 22, p < .0001). All units of mass are given in kg; length is given in meters.
Body Mass at Departure from the Rookery (Both Sexes)
Calculated by using measured rates of mass loss for each animal to extrapolate from date of last weighing (final mass) forward to departure date. For 31 males weighed only at the end of the breeding fast, final mass was extrapolated to departure date using the regression above. For 40 females weighed only near the end of lactation, the corresponding calculation was based on the regression between mass loss per day (y) and final mass (x): y = 1.486 + .01797x (r2 = .52, N = 22, p = .0002). Similar mass estimates could have been obtained for these 40 females (and the 12 above) using standard length to estimate mass loss rate (r2 = .57, N = 22, p < .0001).
Total Mass Loss over the Breeding Season (Males) or the Lactation Period (Females)
Equals estimated arrival/parturition mass minus estimated departure mass for animals weighed two or more times over the season. Females weighed twice over an interval of less than 18 days were excluded from mass loss analyses. Total mass loss expressed as a percentage of arrival/parturition mass was used as an index of the energetic component of RE.
Pup Mass at Birth
Since different pups were initially weighed from 0 to 5 days postpartum, we standardized pup mass by estimating mass at birth. Initial pup mass (kg) was regressed against pup age (days) for 41 pups 0 to 5 days of age, yielding the following linear regression: Initial mass = 36.9 + 2.2 (age); r2 = .29. For pups 0 to 1 day old, birth mass was taken to equal initial mass. For pups 2 to 5 days old, a mass gain of 2.2 kg/day was assumed, starting at day 1; this amount was subtracted from initial mass to obtain estimated birth mass. Since other studies typically report initial mass and since mass gain of pups can be quite variable during the first week (McCann, Fedak, and Harwood 1989), we also report initial mass.
Pup Mass at Weaning
Pups were usually weighed within a few days of weaning. If weighed before weaning, measured mass gain per day for each pup was used to extrapolate from date of final weighing to weaning date. If weighed after weaning, the following equation was used to estimate mass at weaning since weanlings fast and lose mass:
Mass at weaning = Measured mass·(e k·d ),
where k = .00596 and d = number of days between weaning and weighing (modified from Ortiz, Costa, and Le Boeuf 1978; P. M. Morris, pers. comm.). Pups weighed more than 10 days after weaning were excluded from analysis.
Total Pup Mass Gain
Equals estimated mass at weaning minus estimated mass at birth.
Appendix 10.2
Comments on an Outlier for Pup Mass Gain
The outlier was a male pup of a 424 kg, 3-year-old mother ("G22") and deserves special mention because it illustrates some of the problems inherent in interpreting this type of data. Values for this individual lie well outside the expected range for pup mass gain and weaning mass (fig. 10.3g–j). There are at least three possible explanations for this finding: (1) high maternal investment; (2) high efficiency of mass transfer; and/or (3) milk stealing by the pup from other females. G22 gave birth to an exceptionally large pup (10 kg heavier than expected for her size; fig. 10.3e, f), and she lost 41.2% of her parturition mass, the highest value measured for a female. This large mass loss was due primarily to an unusually long lactation period (27 days) for a 3-year-old. Even taking G22's high RE into consideration, however, the pup was weaned about 30 kg heavier than expected. This fact, plus the suspiciously high mass transfer efficiency of 77% (range was 45–67% for the rest), suggests that the pup stole milk from neighbor-
ing females during the nursing period, although this was not observed. For this reason, maternal mass or energy loss is probably a more reliable index of investment than pup mass or energy gain.
References
Alexander, R. D., and G. Borgia. 1979. On the origin and basis of the male-female phenomenon. In Sexual Selection and Reproductive Competition in Insects , ed. M. S. Blum and N. A. Blum, 417–440. New York: Academic Press.
Anderson, S. S., and M. A. Fedak. 1985. Grey seal males: Energetic and behavioural links between size and sexual success. Animal Behaviour 33: 829–838.
———. 1987. Grey seal, Halichoerus grypus , energetics: Females invest more in male offspring. Journal of Zoology, London 211: 667–679.
Bartsh, S. S., S. D. Johnston, and D. B. Siniff. 1992. Territorial behavior and breeding frequency of male Weddell seals (Leptonychotes weddellii ) in relation to age, size, and concentrations of serum testosterone and cortisol. Canadian Journal of Zoology 70: 680–692.
Bell, G. 1980. The costs of reproduction and their consequences. American Naturalist 116: 45–76.
Bell, G., and V. Koufopanou. 1985. The costs of reproduction. Oxford Surveys in Evolutionary Biology 3: 83–131.
Boness, D. J., W. D. Bowen, and O. T. Oftedal. In press. Evidence of a maternal foraging cycle resembling that of otariid seals in a small phocid, the harbor seal. Behavioral Ecology and Sociobiology .
Bowen, W. D., D. J. Boness, and O. T. Oftedal. 1987. Mass transfer from mother to pup and subsequent mass loss by the weaned pup in the hooded seal, Cystophora cristata. Canadian Journal of Zoology 65: 1–8.
Bowen, W. D., O. T. Oftedal, and D. J. Boness. 1992. Mass and energy transfer during lactation in a small phocid, the harbor seal (Phoca vitulina ). Physiological Zoology 65: 844–866.
Bowen, W. D., and W. T. Stobo. 1991. Reproduction in primiparous and young multiparous grey seal females: Is it successful? In Proceedings of the Ninth Biennial Conference on the Biology of Marine Mammals , December 5–9, 1991, Chicago, 9 (Abstract).
Boyce, M. S. 1981. Beaver life-history responses to exploitation. Journal of Applied Ecology 18: 749–753.
Boyd, I. L., and C. D. Duck. 1991. Mass changes and metabolism in territorial male Antarctic fur seals (Arctocephalus gazella). Physiological Zoology 64: 375–392.
Boyd, R., and J. B. Silk. 1983. A method for assigning cardinal dominance ranks. Animal Behaviour 31: 45–58.
Brunton, D. H. 1986. Fatal antipredator behavior of a killdeer. Wilson Bulletin 98: 605–607.
Burley, N. 1988. The differential-allocation hypothesis: An experimental test. American Naturalist 132: 611–628.
Calow, P. 1979. The cost of reproduction—A physiological approach. Biological Review 54: 23–40.
Campagna, C., B. J. Le Boeuf, M. Lewis, and C. Bisioli. 1992. The Fisherian seal: Equal investment in male and female pups in southern elephant seals. Journal of Zoology, London 226: 551–561.
Caro, T. M. 1988. Adaptive significance of play: Are we getting closer? Trends in Ecology and Evolution 3: 50–54.
Carrick, R., S. E. Csordas, S. E. Ingham, and K. Keith. 1962. Studies on the southern elephant seal, Mirounga leonina (L.). IV. Breeding and development. CSIRO Wildlife Research 7: 161–197.
Caswell, H. 1982. Optimal life history and the age-specific costs of reproduction. Journal of Theoretical Biology 98: 519–529.
Charlesworth, B. 1980. Evolution in Age-Structured Populations . Cambridge: Cambridge University Press.
Charlesworth, B., and J. A. León. 1976. The relation of reproductive effort to age. American Naturalist 110: 449–459.
Cherel, Y., J.-P. Robin, and Y. Le Maho. 1988. Physiology and biochemistry of long-term fasting in birds. Canadian Journal of Zoology 66: 159–166.
Clinton, W. L. 1990. Sexual selection and the life-history of male northern elephant seals. Ph.D. dissertation, University of California, Santa Cruz.
Clinton, W. L., and B. J. Le Boeuf. 1993. Sexual selection's effects on male life history and the pattern of male mortality. Ecology 74: 1884–1892.
Clutton-Brock, T. H. 1984. Reproductive effort and terminal investment in iteroparous animals. American Naturalist 123: 212–229.
Clutton-Brock, T. H., S. D. Albon, R. M. Gibson, and F. E. Guinness. 1979. The logical stag: Adaptive aspects of fighting in red deer (Cervus elaphus L.). Animal Behaviour 27: 211–225.
Clutton-Brock, T. H., S. D. Albon, and F. E. Guinness. 1989. Fitness costs of gestation and lactation in wild mammals. Nature 337: 260–262.
Clutton-Brock, T. H., F. E. Guinness, and S. D. Albon. 1982. Red Deer: Behavior and Ecology of Two Sexes . Chicago: University of Chicago Press.
Cody, M. L. 1971. Ecological aspects of reproduction. In Avian Biology , vol. 1, ed. D. S. Farner and J. R. King, 461–512. New York: Academic Press.
Cooper, C. F., and B. S. Stewart. 1983. Demography of northern elephant seals, 1911–1982. Science 219: 969–971.
Costa, D. P., B. J. Le Boeuf, C. L. Ortiz, and A. C. Huntley. 1986. The energetics of lactation in the northern elephant seal. Journal of Zoology, London 209: 21–33.
Cox, C. R. 1983. Reproductive behaviour of sub-adult elephant seals: The cost of breeding. In Behavioral Energetics: The Cost of Survival in Vertebrates , ed. W. P. Aspey and S. I. Lustick, 89–115. Columbus: Ohio State University Press.
Crocker, D. E. 1992. Reproductive effort and age in female northern elephant seals. Master's thesis, University of California, Santa Cruz.
Deutsch, C. J. 1990. Behavioral and energetic aspects of reproductive effort in male northern elephant seals (Mirounga angustirostris ). Ph.D. dissertation, University of California, Santa Cruz.
Deutsch, C. J., M. P. Haley, and B. J. Le Boeuf. 1990. Reproductive effort of male northern elephant seals: Estimates from mass loss. Canadian Journal of Zoology 68: 2580–2593.
Fagen, R. 1981. Animal Play Behavior . London: Oxford University Press.
Fedak, M. A., and S. S. Anderson. 1982. The energetics of lactation: Accurate measurements from a large wild mammal, the grey seal (Halichoerus grypus ). Journal of Zoology, London 198: 473–479.
———. 1987. Estimating the energy requirements of seals from weight changes. In Approaches to Marine Mammal Energetics , ed. A. C. Huntley, D. P. Costa, G. A. J. Worthy, and M. A. Castellini, 205–226. Lawrence, Kan.: Allen Press.
Gadgil, M., and W. H. Bossert. 1970. Life historical consequences of natural selection. American Naturalist 104: 1–24.
Gales, N. J., and H. R. Burton. 1987. Ultrasonic measurement of blubber thickness of the southern elephant seal, Mirounga leonina (Linn.). Australian Journal of Zoology 35: 207–217.
Gittleman, J. L. 1986. Carnivore life-history patterns: Allometric, phylogenetic, and ecological associations. American Naturalist 127: 744–771.
Gittleman, J. L., and S. D. Thompson. 1988. Energy allocation in mammalian reproduction. American Zoologist 28: 863–875.
Glucksman, A. 1974. Sexual dimorphism in mammals. Biological Review of Cambridge Philosophical Society 49: 423–475.
Green, W. C. H. 1990. Reproductive effort and associated costs in bison (Bison bison ): Do older mothers try harder? Behavioral Ecology 1: 148–160.
Green, W. C. H., and A. Rothstein. 1991. Trade-offs between growth and reproduction in female bison. Oecologia 86: 521–527.
Groscolas, R. 1986. Changes in body mass, body temperature and plasma fuel levels during the natural breeding fast in male and female emperor penguins, Aptenodytes forsteri. Journal of Comparative Physiology 156B: 521–527.
Haley, M. P., C. J. Deutsch, and B. J. Le Boeuf. 1991. A method for estimating mass of large pinnipeds. Marine Mammal Science 7: 157–164.
———. In press. Size, dominance and copulatory success in male northern elephant seals, Mirounga angustirostris. Animal Behaviour .
Hamer, K. C., and R. W. Furness. 1991. Age-specific breeding performance and reproductive effort in great skuas, Catharacta skua. Journal of Animal Ecology 60: 693–704.
Hammill, M. O., C. Lydersen, M. Ryg, and T. G. Smith. 1991. Lactation in the ringed seal (Phoca hispida). Canadian Journal of Fisheries and Aquatic Science 48: 2471–2476.
Härkönen, T., and M.-P. Heide-Jørgensen. 1990. Comparative life histories of East Atlantic and other harbour seal populations. Ophelia 32: 211–235.
Hill, S. E. B. 1987. Reproductive ecology of Weddell seals (Leptonychotes weddelli ) in McMurdo Sound, Antarctica. Ph.D. dissertation, University of Minnesota, Minneapolis.
Hirshfield, M. F., and D. W. Tinkle. 1975. Natural selection and the evolution of reproductive effort. Proceedings of the National Academy of Science, USA 72: 2227–2231.
Huber, H. R. 1987. Natality and weaning success in relation to age of first reproduction in northern elephant seals. Canadian Journal of Zoology 65: 1311–1316.
Huber, H. R., A. C. Rovetta, L. A. Fry, and S. Johnston. 1991. Age-specific natality of northern elephant seals at the South Farallon Islands, California. Journal of Mammalogy 72: 525–534.
Iverson, S. J., W. D. Bowen, D. J. Boness, and O. T. Oftedal. 1993. The effect of maternal size and milk energy output on pup growth in grey seals (Halichoerus grypus). Physiological Zoology 66: 61–88.
Kenagy, G. J. 1987. Energy allocation for reproduction in the golden-mantled ground squirrel. Symposium of Zoological Society of London 57: 259–273.
Kenagy, G. J., S. M. Sharbaugh, and K. S. Nagy. 1989. Annual cycle of energy and time expenditure in a golden-mantled ground squirrel population. Oecologia 78: 269–282.
Knapton, R. W. 1984. Parental investment: The problem of currency. Canadian Journal of Zoology 62: 2673–2674.
Kovacs, K. M., and D. M. Lavigne. 1986. Maternal investment and neonatal growth in phocid seals. Journal of Animal Ecology 55: 1035–1051.
Kovacs, K. M., D. M. Lavigne, and S. Innes. 1991. Mass transfer efficiency between harp seal (Phoca groenlandica ) mothers and their pups during lactation. Journal of Zoology, London 223: 213–221.
Kretzmann, M. B. 1990. Maternal investment and the post-weaning fast in northern elephant seals: Evidence for sexual equality. Master's thesis, University of California, Santa Cruz.
Kretzmann, M. B., D. P. Costa, and B. J. Le Boeuf. 1993. Maternal energy investment in elephant seal pups: Evidence for sexual equality? American Naturalist 141: 466–480.
Laws, R. M. 1953. The elephant seal (Mirounga leonina Linn.). I. Growth and age. Falkland Islands Dependencies Survey, Scientific Reports 8: 1–62.
Leader-Williams, N., and C. Ricketts. 1981. Seasonal and sexual patterns of growth and condition of reindeer introduced into South Georgia. Oikos 38: 27–39.
Le Boeuf, B. J. 1974. Male-male competition and reproductive success in elephant seals. American Zoologist 14: 163–176.
———. 1981. Elephant seals. In The Natural History of Año Nuevo , ed. B. J. Le Boeuf and S. Kaza, 326–374. Pacific Grove, Calif.: Boxwood Press.
Le Boeuf, B. J., R. Condit, and J. Reiter. 1989. Parental investment and the secondary sex ratio in northern elephant seals. Behavioral Ecology and Sociobiology 25: 109–117.
Le Boeuf, B. J., D. P. Costa, A. C. Huntley, and S. D. Feldkamp. 1988. Continuous, deep diving in female northern elephant seals, Mirounga angustirostris. Canadian Journal of Zoology 66: 446–458.
Le Boeuf, B. J., D. P. Costa, A. C. Huntley, G. L. Kooyman, and R. W. Davis. 1986. Pattern and depth of dives in northern elephant seals, Mirounga angustirostris. Journal of Zoology, London 208: 1–7.
Le Boeuf, B. J., and S. Kaza, ed. 1981. The Natural History of Año Nuevo . Pacific Grove, Calif.: Boxwood Press.
Le Boeuf, B. J., and S. Mesnick. 1991. Sexual behavior of male northern elephant seals. I. Lethal injuries to adult females. Behaviour 116: 143–162.
Le Boeuf, B. J., Y. Naito, A. C. Huntley, and T. Asaga. 1989. Prolonged, continuous, deep diving by northern elephant seals. Canadian Journal of Zoology 67: 2514–2519.
Le Boeuf, B. J., and J. Reiter. 1988. Lifetime reproductive success in northern
elephant seals. In Reproductive Success: Studies of Individual Variation in Contrasting Breeding Systems , ed. T. H. Clutton-Brock, 344–362. Chicago: University of Chicago Press.
Le Boeuf, B. J., M. Riedman, and R. S. Keyes. 1982. White shark predation on pinnipeds in California coastal waters. Fishery Bulletin 80: 891–895.
Lee, A. K., P. Wooley, and R. W. Braithewaite. 1982. Life history strategies of dasyurid marsupials. In Carnivorous Marsupials , vol. 1, ed. M. Archer, 1–11. Chipping Norton, NSW: Surry Beatty and Sons.
Lewontin, R. C. 1965. Selection for colonizing ability. In The Genetics of Colonizing Species , ed. H. G. Baker and G. L. Stebbins, 77–91. New York: Academic Press.
Loudon, A. S. I., and P. A. Racey, eds. 1987. Reproductive Energetics of Mammals . Symposia of the Zoological Society of London no. 57. Oxford: Oxford University Press.
Low, B. S. 1978. Environmental uncertainties and the parental strategies of marsupials and placentals. American Naturalist 112: 197–213.
McCann, T. S., M. A. Fedak, and J. Harwood. 1989. Parental investment in southern elephant seals, Mirounga leonina. Behavioral Ecology and Sociobiology 25: 81–87.
Maher, C. R., and J. A. Byers. 1987. Age-related changes in reproductive effort of male bison. Behavioral Ecology and Sociobiology 21: 91–96.
Maynard Smith, J. 1980. A new theory of sexual investment. Behavioral Ecology and Sociobiology 7: 247–251.
Mesnick, S., and B. J. Le Boeuf. 1991. Sexual behavior of male northern elephant seals. II. Female responses to potentially fatal mating encounters. Behaviour 117: 262–280.
Michener, G. R., and L. Locklear. 1990. Differential costs of reproductive effort for male and female Richardson's ground squirrels. Ecology 71: 855–868.
Millar, J. S. 1977. Adaptive features of mammalian reproduction. Evolution 31: 370–386.
Nur, N. 1984. Increased reproductive success with age in the California gull: Due to increased effort or improvement of skill? Oikos 43: 407–408.
Oftedal, O. T., D. J. Boness, and R. A. Tedman. 1987. The behavior, physiology, and anatomy of lactation in the Pinnipedia. Current Mammalogy 1: 175–245.
Oftedal, O. T., S. J. Iverson, and D. J. Boness. 1987. Milk and energy intakes of suckling California sea lion, Zalophus californianus , pups in relation to sex, growth, and predicted maintenance requirements. Physiological Zoology 60: 560–575.
Ortiz, C. L., D. P. Costa, and B. J. Le Boeuf. 1978. Water and energy flux in elephant seal pups fasting under natural conditions. Physiological Zoology 51: 166–178.
Ortiz, C. L., B. J. Le Boeuf, and D. P. Costa. 1984. Milk intake: An index of maternal investment in elephant seal pups. American Naturalist 124: 416–422.
Packard, G. C., and T. J. Boardman. 1987. The misuse of ratios to scale physiological data that vary allometrically with body size. In New Directions in Ecological Physiology , ed. M. E. Feder, A. F. Bennett, W. W. Burggren, and R. B. Huey, 216–239. Cambridge: Cambridge University Press.
Pärt, T., L. Gustafsson, and J. Moreno. 1992. "Terminal investment" and a sexual conflict in the collared flycatcher (Ficedula albicollis). American Naturalist 140: 868–882.
Pianka, E. R. 1976. Natural selection of optimal reproductive tactics. American Zoologist 16: 775–784.
Pianka, E. R., and W. S. Parker. 1975. Age-specific reproductive tactics. American Naturalist 109: 453–464.
Pugesek, B. H. 1981. Increased reproductive effort with age in the California gull (Larus californicus). Science 212: 822–823.
———. 1983. The relationship between parental age and reproductive effort in the California gull. Behavioral Ecology and Sociobiology 13: 161–171.
———. 1984. Age-specific reproductive tactics in the California gull. Oikos 43: 409–410.
Rasa, O. A. E. 1971. Social interaction and object manipulation in weaned pups of the northern elephant seal Mirounga angustirostris. Zeitschift Tierpsychologie 29: 82–102.
Reid, W. V. 1988. Age-specific patterns of reproduction in the glaucous-winged gull: Increased effort with age? Ecology 69: 1454–1465.
Reilly, J. J. 1989. The water and energy metabolism of breeding male grey seals (Halichoerus grypus ). In Proceedings of the Eighth Biennial Conference on the Biology of Marine Mammals , December 7–11, 1989, Pacific Grove, Calif., 53 (Abstract).
Reilly, J. J., and M. A. Fedak. 1991. Rates of water turnover and energy expenditure of free-living male common seals (Phoca vitulina). Journal of Zoology, London 223: 461–468.
Reiter, J., and B. J. Le Boeuf. 1991. Life history consequences of variation in age at primiparity in northern elephant seals. Behavioral Ecology and Sociobiology 28: 153–160.
Reiter, J., K. J. Panken, and B. J. Le Boeuf. 1981. Female competition and reproductive success in northern elephant seals. Animal Behaviour 29: 670–687.
Reiter, J., N. L. Stinson, and B. J. Le Boeuf. 1978. Northern elephant seal development: The transition from weaning to nutritional independence. Behavioral Ecology and Sociobiology 3: 337–367.
Rice, W. R., and S. D. Gaines. 1989. One-way analysis of variance with unequal variances. Proceedings of the National Academy of Sciences, USA 86: 8183–8184.
———. 1993. Calculating p-values for ANOVA with unequal variances. Journal of Statistical Computation and Simulation 46: 19–22.
Riedman, M. 1990. The Pinnipeds: Seals, Sea Lions, and Walruses . Berkeley, Los Angeles, and Oxford: University of California Press.
Robbins, C. T., and B. L. Robbins. 1979. Fetal and neonatal growth patterns and maternal reproductive effort in ungulates and subungulates. American Naturalist 114: 101–116.
Robinson, B. W., and R. W. Doyle. 1985. Trade-off between male reproduction (amplexus) and growth in the amphipod Gammarus lawrencianus. Biological Bulletin 168: 482–488.
Robinson, S. K. 1988. Anti-social and social behaviour of adolescent yellow-rumped caciques (Icterinae: Cacicus cela). Animal Behaviour 36: 1482–1495.
Ryan, M. J. 1985. The Túngara Frog: A Study in Sexual Selection and Communication . Chicago: University of Chicago Press.
Sandegren, F. E. 1976. Agonistic behavior in the male northern elephant seal. Behaviour 57: 136–158.
SAS Institute. 1985. SAS User's Guide: Statistics . Ver. 5. Cary, N.C.: SAS Institute.
Selander, R. K. 1972. Sexual selection and dimorphism in birds. In Sexual Selection and the Descent of Man, 1871–1971 , ed. B. G. Campbell, 180–230. Chicago: Aldine.
Sergeant, D. E. 1973. Feeding, growth, and productivity of Northwest Atlantic harp seals (Pagophilus groenlandicus). Journal of Fisheries Research Board of Canada 30: 17–29.
Sibly, R., and P. Calow. 1986. Why breeding earlier is always worthwhile. Journal of Theoretical Biology 123: 311–319.
Smith, M. S. R. 1966. Injuries as an indication of social behaviour in the Weddell seal (Leptonychotes weddelli). Mammalia 30: 241–246.
Sokal, R. R., and F. S. Rohlf. 1981. Biometry . San Francisco: W. H. Freeman and Co.
Stearns, S. C. 1976. Life history tactics: A review of the ideas. Quarterly Review of Biology 51: 3–47.
Stewart, R. E. A. 1986. Energetics of age-specific reproductive effort in female harp seals, Phoca groenlandica. Journal of Zoology, London (A) 208: 503–517.
Stewart, R. E. A., and D. M. Lavigne. 1984. Energy transfer and female condition in nursing harp seals, Phoca groenlandica. Holarctic Ecology 7: 183–194.
Sydeman, W. J., H. R. Huber, S. D. Emslie, C. A. Ribic, and N. Nur. 1991. Age-specific weaning success of northern elephant seals in relation to previous breeding experience. Ecology 72: 2204–2217.
Tedman, R., and B. Green. 1987. Water and sodium fluxes and lactational energetics in suckling pups of Weddell seals (Leptonychotes weddellii). Journal of Zoology, London 212: 29–42.
Testa, J. W., S. E. B. Hill, and D. B. Siniff. 1989. Diving behavior and maternal investment in Weddell seals (Leptonychotes weddellii). Marine Mammal Science 5: 399–405.
Thornhill, R. 1981. Panorpa (Mecoptera: Panorpidae) scorpionflies: Systems for understanding resource-defense polygyny and alternative male reproductive efforts. Annual Review of Ecology and Systematics 12: 355–386.
Trivers, R. L. 1972. Parental investment and sexual selection. In Sexual Selection and the Descent of Man, 1871–1971 , ed. B. Campbell, 136–179. Chicago: Aldine.
———. 1985. Social Evolution . Menlo Park, Calif.: Benjamin/Cummings Publishing Co.
Tuomi, J., T. Hakala, and E. Haukioja. 1983. Alternative concepts of reproductive effort, costs of reproduction, and selection in life-history evolution. American Zoologist 23: 25–34.
Twiss, S. D. 1991. Behavioural and energetic determinants of individual mating success in male grey seals (Halichoerus grypus , Fabricius 1791). Ph.D. thesis, University of Glasgow, Scotland.
Walker, B. G., and W. D. Bowen. In press. Changes in body mass and feeding behaviour in male harbour seals, Phoca vitulina , in relation to female reproductive status. Journal of Zoology, London .
Warner, R. R. 1980. The coevolution of life-history and behavioral characteristics. In Sociobiology: Beyond Nature/Nurture? ed. G. W. Barlow and J. S. Silverberg, 151–188. Boulder, Colo.: Westview Press.
Wilkinson, P. F., and C. C. Shank. 1976. Rutting-fight mortality among musk oxen
on Banks Island, Northwest Territories, Canada. Animal Behaviour 24: 756–758.
Williams, G. C. 1966a . Natural selection, the costs of reproduction, and a refinement of Lack's principle. American Naturalist 100: 687–690.
———. 1966b. Adaptation and Natural Selection . Princeton: Princeton University Press.
Worthy, G. A. J., P. A. Morris, D. P. Costa, and B. J. Le Boeuf. 1992. Moult energetics of the northern elephant seal. Journal of Zoology, London 227: 257–265.
Eleven—
Diet of the Northern Elephant Seal
George A. Antonelis, Mark S. Lowry, Clifford H. Fiscus, Brent S. Stewart, and Robert L. DeLong
ABSTRACT. The diet of northern elephant seals, Mirounga angustirostris , at San Miguel Island, California, was examined by stomach lavage during the spring and summer from 1984 through 1990. Identifiable hard parts of prey species were recovered from the stomachs of 193 of 195 seals sampled. Cephalopods occurred in all stomachs containing identifiable remains of prey. Most prey species (70%) identified inhabit epi-, meso-, or bathypelagic oceanic zones, and relatively few (30%) occur in neritic or benthic regions. The five most frequently occurring prey species were the cephalopods Octopoteuthis deletron (58.0%), Histioteuthis heteropsis (43.0%), Gonatopsis borealis (40.9%), Histioteuthis dofleini (39.4%) and the teleost Merluccius productus (38.9%). The diets of males and females were similar, with the exception of a significantly higher occurrence of cyclostomes in the stomachs of subadult males. Variability in the diet of elephant seals was probably influenced by annual changes in the availability and abundance of prey.
The population of northern elephant seals, Mirounga angustirostris , has increased greatly during the past eight decades and may now number about 127,000 rangewide (Bartholomew and Hubbs 1960; Cooper and Stewart 1983; Stewart et al., this volume). Elephant seal behavior during brief periods ashore has been well documented (e.g., Bartholomew 1952; Le Boeuf 1974; Reiter, Panken, and Le Boeuf 1981), but their foraging ecology has only recently come under study (Hacker 1986; Antonelis et al. 1987; Le Boeuf et al. 1988, 1989; DeLong and Stewart 1991; Sakamoto et al. 1989; DeLong, Stewart, and Hill 1992; Le Boeuf, this volume; Stewart and DeLong, this volume). Here we describe the diet of northern elephant seals based on the examination of prey remains recovered from stomach contents and discuss the ecological relationships between elephant seals and their prey.
Methods
We lavaged the stomachs of 11 adult male, 69 subadult male, and 115 female northern elephant seals when they hauled out on land to molt at San Miguel Island, California, in spring (late April/early May) and summer (July) from 1984 through 1990. Stomach lavage was accomplished by chemically immobilizing each seal with ketamine hydrochloride, intubating its stomach, and flushing out the remaining parts of prey with 3 to 5 liters of seawater (Antonelis et al. 1987; DeLong and Stewart 1991). Prolonged apnea associated with the use of ketamine hydrochloride and poor health condition resulted in the death of 6 of 195 elephant seals chemically immobilized. Portions of the dietary information from the 1984 field season were previously reported by G. A. Antonelis et al. (1987).
The percent occurrence of prey species found in the stomachs of elephant seals was used as an index of prey consumption. Those species occurring in over 30% of the stomachs were considered major prey items. Dietary information was determined by identifying remains of sagittal otoliths and bones (fish), mouthparts (cephalopods and cyclostomes), elasmobranch and cyclostome egg cases (Cox 1963), crustacean exoskeletons (Schmitt 1921), and tunicates (Thompson 1948).
We estimated age groups (< 1 yr., 1 yr., 2 yrs., 3 yrs., 4–7 yrs.) of Pacific whiting, Merluccius productus , eaten by seals in 1984–1986 on the basis of size relationships between otoliths from stomach contents and known-age whiting reference specimens (Antonelis et al. 1987) and then estimated fish size using relationships among age, weight, and length (Dark 1975). Digestive erosion did not compromise our ability to categorize otoliths into age groups because Pacific Whiting size differences are conspicuous during the first three years of rapid growth (Dark 1975; Antonelis et al. 1987).
We estimated body sizes (mantle length) of individuals of one cephalopod prey, Gonatopsis borealis , from measurements of lower rostral beak length (Clarke 1986). These beak measurements were obtained from samples collected from 1984 to 1986. Sufficient data on relationships between body size and beak measurements were not available for the other cephalopods identified in this study. The annual occurrence of single and multiple prey taxa found in the stomachs of elephant seals (1984–1986) was calculated as percentages of the total number of stomachs containing identifiable food remains.
The null hypotheses (1) male and female elephant seals consumed the same prey and (2) diet did not differ among years were tested with a logistic regression analysis model (Aitkin et al. 1989). We compared the deviance explained by the variables (sex and year) to the deviance of the model without entering the variables. Changes in deviance were approximated by a chi-square distribution with degrees of freedom equal to the change in the
degrees of freedom between the two models. Data from stomach lavages conducted from 1988 to 1990 were not tested statistically because of insufficient sample sizes.
Results
We identified 53 prey taxa from stomach contents of 193 elephant seals (tables 11.1 and 11.2). No identifiable remains of prey were recovered from the stomachs of two subadult males. Thirty-seven (70%) of those taxa inhabit one or more of the pelagic habitats (epi-, meso-, or bathypelagic), whereas 16 taxa (30%) are restricted to neritic or benthic zones.
Cephalopods were the most commonly identified prey of elephant seals (table 11.1), consisting of 28 species, 6 of which have not been previously reported as prey of northern elephant seals (Gonatus onyx, Japetella heathi, Architeuthis japonica, Megalocranchia sp., Octopus bimaculatus , and Ommastrephes bartrami ). We recovered cephalopod remains from all of the stomachs containing identifiable parts of prey species (11 adult males, 67 subadult males, and 115 adult females). Octopoteuthis deletron, Histioteuthis heteropsis, Gonatopsis borealis , and Histioteuthis dofleini were the most frequently occurring cephalopods found in the stomachs. We estimated mantle length of G. borealis eaten as 13.6–28.0 cm

Other prey groups were recovered from stomachs less often than cephalopods. Pacific whiting and pelagic red crabs, Pleuroncodes planipes , were the predominant fish and crustacean species (table 11.2). Two of the identified fish species, Sebastolobus alascanus and Icichythys lockingtoni , had not been previously reported as elephant seal prey.
Diets of male and female seals were not significantly different (c2 > 3.84, df = 1, p > .05) with one exception; cyclostomes occurred more frequently in the stomachs of subadult males (c2 = 3.84, df = 1, p < .05) than in adult females (fig. 11.1). Seals ate teleost fish more frequently (c2 = 5.99, df = 2, p < .05) in 1985 (males = 42%, females = 54%) than they did in 1986 (males = 30%, females = 27%). Crustaceans were consumed more often (c2 = 5.99, df = 2, p < .05) in 1984 (males = 80%, females = 76%) than in 1986 (males = 17%, females = 14%); cephalopods were eaten more frequently (c 2 = 5.99, df = 2, p < .05) in 1985 (males = 95%, females = 97%) than in 1984 (males = 76%, females = 79%). The diversity of prey eaten varied among years, with the greatest number of taxa consumed in 1984 and the fewest in 1986 (fig. 11.2).
The age composition of Pacific whiting that were eaten by elephant seals varied among years (fig. 11.3), but most fish (79% of 252) were less than 4 years old. Overall, 2-year-old Pacific whiting were the most often eaten age group (33%), followed by 1-year-old (21%), 4- to 7-year-old (21%), 3-year-
|
|
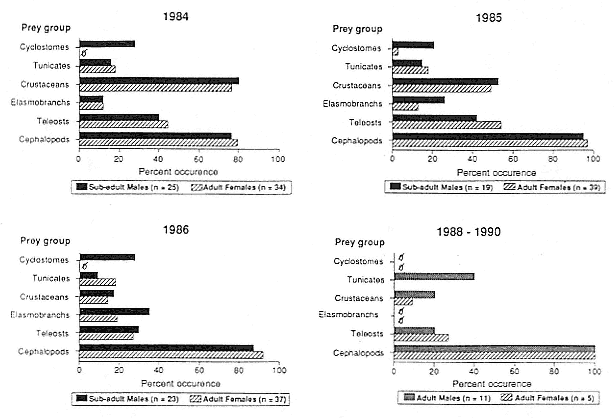
Fig. 11.1
Percent occurrence of the six major prey categories lavaged from the stomachs of adult male,
subadult male, and adult female northern elephant seals (1984, 1985, 1986, and 1988–1990).
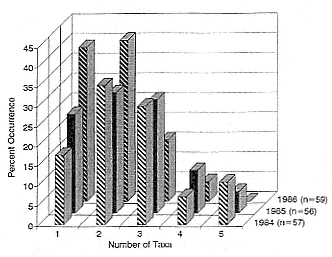
Fig. 11.2
Occurrence of single and multiple prey taxa lavaged from
the stomachs of northern elephant seals, 1984–1986.
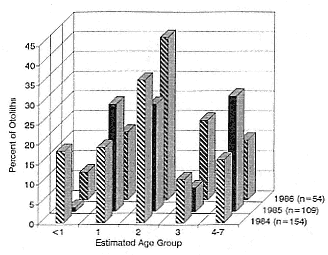
Fig. 11.3
Estimated age of Pacific whiting otoliths lavaged from the
stomachs of northern elephant seals, 1984–1986.
old (15%), and first-year fish (10%). Pacific whiting that were less than 1 year old were rarely eaten in 1985 (1%) and in 1986 (7%) but were common prey (20%) in 1984 (fig. 11.3). The 1984 Pacific whiting cohort was also well represented in the diets of seals as 1-year-old fish in 1985 (27%) and as 2-year-old fish in 1986 (41%). The frequency of occurrence of 3-and 4-year-old Pacific whiting was greatest in 1986 and 1985, respectively (fig. 11.3).
The average length and weight estimates of individual 1-year-old, 2-year-old, and 3-year-old Pacific whiting were 15.4 cm and 0.038 kg, 28.0 cm and 0.163 kg, and 42.3 cm and 0.496 kg, respectively. The average size of individual 4- to 7-year-old Pacific whiting ranged from 44.6 cm (~ 0.570 kg) to 51.8 cm (~ 0.854 kg).
Discussion
Our lavage studies indicate that elephant seals feed primarily on epi- and mesopelagic cephalopods, although other prey types that inhabit pelagic habitats are occasionally eaten. Recent data on the spatial and temporal distribution of foraging adult northern elephant seals (Stewart and DeLong 1990; Le Boeuf, this volume; Stewart and DeLong, this volume) reinforce these interpretations of the importance of resources in meso- and epipelagic habitats to northern elephant seals. Benthic organisms in the neritic zone may be more important prey for adult elephant seals when they forage over the continental shelf during migrations to and from breeding or haul-out locations. The foraging locations of juvenile elephant seals are unknown, but studies are currently under way (Le Boeuf, this volume). Limited dietary information (Hacker 1986) indicates that juveniles may feed frequently in the neritic zone.
All five major prey species make some type of diel vertical migration. O. deletron has been described as a vertical spreader that is found at 200 to 400 m depths during the day but disperses both upward (usually in the upper 100 m at night) and downward at night; the maximum reported depth is 1,600 m (Jefferts 1983; Roper and Young 1975). H. heteropsis, H. dofleini , and G. borealis are all second-order vertical migrators that move toward the surface at night (usually no shallower than 200 m) from daytime depths of 300 to 400 m (H. heteropsis and H. dofleini ) to 400 to 700 m (G. borealis ; Jefferts 1983, Roper and Young 1975). Pacific whiting migrate between daytime depths of 150 to 200 m and surface waters at night (Ermakov 1974); maximum daytime depths may reach 1,000 m (Best 1963). These depth ranges and diel variations of prey distribution are consistent with data on diving patterns recorded for adult elephant seals (e.g., Le Boeuf et al. 1986, 1988, 1989; DeLong and Stewart 1991; Stewart and DeLong, this volume; Le Boeuf, this volume). These results are also consistent
with the hypothesis that adult female and adult male seals dive to and forage on vertically migrating prey in the offshore mesopelagic zone (Le Boeuf et al. 1988; DeLong and Stewart 1991). Additional studies of the diel movement patterns of these prey and their association with the deep scattering layer may provide valuable insight into the foraging behavior of the northern elephant seal.
The luminescent characteristics of cephalopods may be an important factor that facilitates their being visually detected and preyed on by elephant seals, especially under low-light conditions. Three of the four major cephalopod prey (O. deletron, H. heteropsis , and H. dofleini ) are highly luminescent relative to the other less frequently occurring cephalopod prey (Nesis 1982). Unlike most of the other prey, these three cephalopods occur in the darkness of the bathypelagic zone, where a high degree of luminescence might make them more vulnerable to predation by elephant seals.
Only two other prey occurred in over 30% of the stomachs of elephant seals: pelagic red crabs (P. planipes ) and Pacific whiting. Pelagic red crabs were transient prey, occurring in the diet of the seals only in 1984 (44%) and in 1985 (38%). Pelagic red crabs have rarely been recovered during lavage studies since this time. Their consumption was evidently linked to the movement of large numbers of these crabs into the offshore waters of California during the 1982–83 El Niño Southern Oscillation (Stewart, Yochem, and Schreiber 1984; Hacker 1986). The influx of this prey into the region and, perhaps, the change in availability of other prey (e.g., Bailey and Incze 1985; Fiedler, Methot, and Hewitt 1986; Trillmich and Ono 1991) likely contributed to the high frequency of occurrence of P. planipes in those years.
The variable patterns of occurrence of Pacific whiting in the diet of northern elephant seals illustrate the relationship between the abundance and availability of a prey resource and the frequency of its consumption (fig. 11.3). Juvenile Pacific whiting (<1–3 years of age) are most commonly found in the epi- and mesopelagic waters, near the central and southern California coasts (Bailey, Francis, and Stevens 1982). Biomass estimates of this resource vary annually and are greatly influenced by the strength of each year class (Dorn et al. 1990). The great strength of the 1984 whiting cohort (ibid.) was clearly reflected in the seals' diets. Relative to other cohorts, the 1984 cohort occurred most often in elephant seals' diets each year as first-year (1984), 1-year-old (1985), and 2-year-old fish (1986). Similar relationships have been demonstrated between the abundance and availability of prey resources and their consumption by California sea lions, Zalophus californianus (Bailey and Ainley 1982; Antonelis, Fiscus, and DeLong 1984; Lowry et al. 1990).
Most other noncephalopod prey were only occasionally eaten during the spring and summer months. The inclusion of these prey in the diet of the
northern elephant seal does indicate, however, that these seals are capable of foraging on other prey species. For example, when resource availability was altered by the 1982–1983 El Niño Southern Oscillation (Trillmich and Ono 1991), the effects continued through 1984 when elephant seals foraged on a greater variety of prey taxa. G. A. Antonelis and C. H. Fiscus (1980) suggested that such dietary diversification might be expected during times of resource depletion.
The only detectable difference between the diets of subadult males and adult females was the more frequent occurrence of cyclostomes in the diet of the former. E. S. Hacker (1986) reported that juvenile males and females ate cyclostomes as well as other neritic and nearshore benthic animals. Further studies are needed to determine whether or not consistent differences exist between adult and immature elephant seals.
Northern elephant seals and other pinnipeds in the California Current eat some of the same prey (Antonelis and Fiscus 1980). The degree to which their foraging habitats overlap is minimized by the relatively short time elephant seals remain in nearshore waters. Most adult males and females move far offshore of their rookeries to feed in the Gulf of Alaska and the central/eastern North Pacific, respectively (Stewart and DeLong 1990; Le Boeuf, this volume; Stewart and DeLong, this volume). The degree to which juveniles forage in nearshore waters is poorly understood.
The size of prey consumed by northern elephant seals is not well documented. Our limited results on the size of Pacific whiting and G. borealis indicate that elephant seals tend to forage on prey that range from about 13 to 52 cm. O. deletron, H. heteropsis , and H. dofleini also occur in this size range (Nesis 1982), but additional information is needed to accurately describe the size of these and other prey consumed by northern elephant seals.
Assessing the importance of prey species on the basis of trace remains obtained by lavaging the stomachs of elephant seals must always be made with caution. Some of these prey, such as euphausiids, may be eaten incidental to other prey or may be secondary prey items (prey of the primary prey species) as suggested by W. F. Perrin et al. (1973). Biases may result also from individual differences in the time between feeding and lavage. In studies such as ours, most of the prey species of northern elephant seals are probably consumed relatively close to San Miguel Island and do not adequately represent those prey eaten while on the foraging grounds in the Gulf of Alaska or the central/eastern North Pacific (Stewart and DeLong 1990; Le Boeuf, this volume; Stewart and DeLong, this volume). Differential passage rates of cephalopod beaks versus otoliths in the stomachs of marine predators is another possible source of bias (Fiscus 1990; Miller 1978; Pitcher 1980). Such biases make it extremely difficult to accurately evaluate the energetic contribution of various prey species to the diet of northern elephant seals. Despite these limitations, we have demonstrated
the ability to detect changes in the diet of elephant seals that are associated with documented changes in the availability of prey (e.g., Pacific whiting and pelagic red crabs). Similar predator-prey relationships cannot be made for cephalopods and elephant seals because so little information is available on the ecology and status of cephalopod stocks in the North Pacific. This lack of information emphasizes the need for future studies on the ecological relationships between northern elephant seals and their prey.
Acknowledgments
We thank the following for their aid in this study: E. Berry, J. Barlough, D. DeMaster, S. Diamond, D. Hanan, S. Hawes, T. Loughlin, L. Hansen, J. Scholl, and D. Skilling for field assistance; J. Allen, S. Crockford, J. Dunn, D. Dwyer, B. Goetz, S. Hawes, F. G. Hochberg, D. Siefert, R. Wigen, and M-S. Yang for identifying prey remains; S. Melin for data base organization; B. Sinclair for assisting during the analysis of prey remains and for reviewing literature; A. York and L. Fore for statistical advice; and the staffs of the National Marine Mammal Laboratory, Alaska Fisheries Science Center, and Coastal Zone and Estuarine Studies Division Development Shop, Northwest Fisheries Science Center, Seattle, for help in constructing the lavage apparatus. J. Baker, B. Le Boeuf, R. Gentry, G. Kooyman, T. Loughlin, M. Perez, and B. Sinclair provided helpful critical reviews of the manuscript.
References
Aitkin, M., D. Anderson, B. Francis, and J. Hinde. 1989. Statistical Modeling in GLIM . Oxford: Clarendon Press.
Antonelis, G. A., and C. H. Fiscus. 1980. The pinnipeds of the California current. California Cooperative Oceanic Fisheries Investigations Report 21: 68–78.
Antonelis, G. A., C. H. Fiscus, and R. L. DeLong. 1984. Spring and summer prey of California sea lions, Zalophus californianus , at San Miguel Island, California, 1978–1979. Fishery Bulletin 82: 67–76.
Antonelis, G. A., M. S. Lowry, D. P. DeMaster, and C. H. Fiscus. 1987. Assessing northern elephant seal feeding habits by stomach lavage. Marine Mammal Science 3: 308–322.
Bailey, K. M., and D. G. Ainley. 1982. The dynamics of California sea lion predation of Pacific whiting. Fishery Research 1: 163–176.
Bailey, K. M., R. C. Francis, and P. R. Stevens. 1982. The life history and fishery of Pacific whiting, Merluccius productus. California Cooperative Oceanic Fisheries Investigations Report 23: 81–98.
Bailey, K. M., and L. S. Incze. 1985. El Niño and the early life history of recruitment of fishes in temperate marine waters. In El Niño North , ed. W. S. Wooster
and D. L. Fluharty, 143–165. Washington Sea Grant, University of Washington, Seattle.
Bartholomew, G. A. 1952. Reproductive and social behavior of the northern elephant seal. University of California Publications in Zoology 47: 369–472.
Bartholomew, G. A., and C. L. Hubbs. 1960. Population growth and seasonal movements of the northern elephant seal, Mirounga angustirostris. Mammalia 24: 313–324.
Best, E. A. 1963. Contribution to the biology of Pacific hake, Merluccius productus. California Cooperative Oceanic Fisheries Investigations Report 9: 51–56.
Butler, T. H. 1980. Shrimps of the Pacific coast of Canada. Canadian Bulletin of Fisheries and Aquatic Sciences 202.
Clarke, M. R., ed. 1986. A Handbook for the Identification of Cephalopod Beaks . Oxford: Clarendon Press.
Cooper, C. F., and B. S. Stewart. 1983. Demography of northern elephant seals, 1911–1982. Science 219: 969–971.
Cox, K. W. 1963. Egg-cases of some elasmobranchs and a cyclostome from California waters. California Fish and Game 49: 271–289.
Dark, T. A. 1975. Age and growth of Pacific hake, Merluccius productus. Fishery Bulletin 73: 336–355.
DeLong, R. L., and B. S. Stewart. 1991. Diving patterns of northern elephant seal bulls. Marine Mammal Science 7: 369–384.
DeLong, R. L., B. S. Stewart, and R. D. Hill. 1992. Documenting migrations of northern elephant seals using day length. Marine Mammal Science 8: 155–159.
Dorn, M. W., R. D. Methot, E. P. Nunnallee, and M. E. Wilkins. 1990. Status of the coastal Pacific whiting resource in 1990. Status of the Pacific coast groundfish fishery through 1990 and recommended acceptable biological catches for 1991. Stock assessment and fishery evaluation. Appendix Vol. I. Pacific Fishery Management Council, Portland, Ore.
Ermakov, Y. K. 1974. The biology and fishery of Pacific hake, Merluccius productus . Ph.D. dissertation, Pacific Scientific Institute of Marine Fisheries and Oceanography (TINRO), Vladivostok, USSR.
Fiedler, P. C., R. D. Methot, and R. P. Hewitt. 1986. Effects of California El Niño 1982–1984 on the northern anchovy. Journal of Marine Research 44: 317–338.
Fiscus, C. H. 1990. Notes on North Pacific gonatids: Identification of body fragments and beaks from marine mammals. Abstracts and Proceedings of the Annual Meeting held in Seattle, Wash., June 18–22, 1990, Western Society of Malacologists, Annual Report 23.
Hacker, E. S. 1986. Stomach content analysis of short-finned pilot whales (Globicephala macrorhynchus ) and northern elephant seals (Mirounga angustirostris ) from the southern California Bight. National Marine Fisheries Service, Southwest Fisheries Center Administrative Report LJ-86-08C.
Jefferts, K. 1983. Zoogeography and systematics of cephalopods of the northeastern Pacific Ocean. Ph.D. dissertation, Oregon State University, Corvallis.
Le Boeuf, B. J. 1974. Male-male competition and reproductive success in elephant seals. American Zoologist 14: 163–176.
Le Boeuf, B. J., D. P. Costa, A. C. Huntley, and S. D. Feldkamp. 1988. Continuous,
deep diving in female northern elephant seals, Mirounga angustirostris. Canadian Journal of Zoology 66: 446–458.
Le Boeuf, B. J., D. P. Costa, A. C. Huntley, G. L. Kooyman, and R. W. Davis. 1986. Pattern and depth of dives in northern elephant seals, Mirounga angustirostris. Journal of Zoology, London 208: 1–7.
Le Boeuf, B. J., Y. Naito, A. C. Huntley, and T. Asaga. 1989. Prolonged, continuous, deep diving by northern elephant seals. Canadian Journal of Zoology 67: 2514–2519.
Lowry, M. S., C. W. Oliver, C. Macky, and J. B. Wexler. 1990. Food habits of California sea lions, Zalophus californianus , at San Clemente Island, California. Fishery Bulletin 88: 509–521.
Miller, D. S., and R. N. Lea. 1972. Guide to the coastal marine fishes of California. California Department of Fish and Game, Fish Bulletin 157: 1–235.
Miller, L. K. 1978. Energetics of the Northern Fur Seal in Relation to Climatic and Food Resources of the Bering Sea . Marine Mammal Commission, Washington, D.C. (Available U.S. Department of Commerce, National Technical Information Service, as PB-275296.)
Nesis, K. N. 1982. Cephalopods of the World . Translated from Russian by B. S. Levitov and edited by L. A. Burgess. Department of Nekton, P. P. Shirshov Institute of Oceanography, USSR Academy of Sciences, Moscow.
Perrin, W. F., R. R. Warner, C. H. Fiscus, and D. B. Holts. 1973. Stomach contents of porpoise, Stenella spp. and yellowfin tuna, Thunnus albacares , in mixed-species aggregations. Fishery Bulletin 71: 1077–1092.
Pitcher, K. W. 1980. Stomach contents and feces as indicators of harbor seal, Phoca vitulina , foods in the Gulf of Alaska. Fishery Bulletin 78: 797–798.
Reiter, J., K. J. Panken, and B. J. Le Boeuf. 1981. Female competition and reproductive success in northern elephant seals. Animal Behaviour 29: 670–687.
Roper, C. F. E., and R. E. Young. 1975. Vertical distribution of pelagic cephalopods. Smithsonian Contributions to Zoology 209: 1–51.
Sakamoto, W., Y. Naito, A. C. Huntley, and B. J. Le Boeuf. 1989. Daily gross energy requirements of a female northern elephant seal, Mirounga angustirostris. Nippon Suisan Gakkaishi 55: 2057–2063.
Schmitt, W. L. 1921. The marine decapod crustacea of California. University of California Publications in Zoology 23: 1–470.
Stewart, B. S., and R. L. DeLong, 1990. Sexual differences in migrations and foraging behavior of northern elephant seals. American Zoologist 30: 44A.
Stewart, B. S., P. K. Yochem, and R. W. Schreiber. 1984. Pelagic red crabs as a food source for gulls: A possible benefit of El Niño. Condor 86: 341–342.
Thompson, H. 1948. Pelagic tunicates of Australia . Commonwealth Council for Scientific and Industrial Research, Melbourne, Australia.
Trillmich, F., and K. Ono, eds. 1991. Pinnipeds and El Niño . Heidelberg and Berlin: Springer Verlag.