2.2—
Chemical Composition of Membranes
Biological membranes are composed primarily of two main classes of compounds, lipids and proteins, which interact in several ways with water to bring
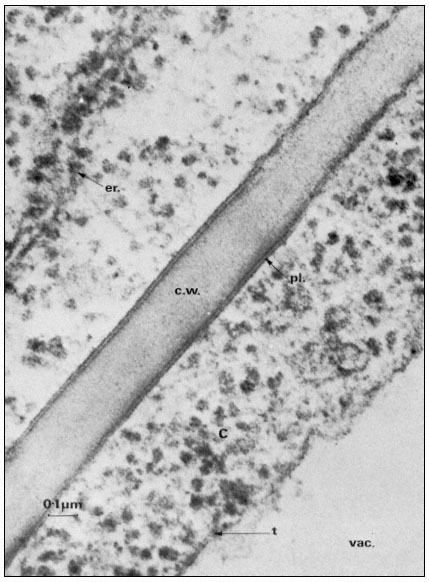
Figure 2.1
Electronmicrograph showing the plasmalemma of two endodermal cells
separated by a cell wall (c.w.) in the root of barley. The plasmalemma (pl.) is
prominently stained in the central region of the picture and is clearly trilaminar.
Notice that the tonoplast (t) bounding the vacuole (vac.) is much less distinct
but also appears trilaminar at the point indicated by the arrow. Other symbols
er = endoplasmic reticulum; c = cytoplasm. Total magnification about × 200,000.
(Micrograph by courtesy of Dr. A. W. Robards).
about a characteristic trilaminar structure. As a very broad generalization it might be said that lipids make up the supporting matrix of the membrane while the proteins thus supported determine its specific properties. This is, of course, merely a convenient simplification as it is becoming plain that some of the characteristic properties of a membrane, particularly those which determine its effectiveness as a barrier to the diffusion of water and electrolytes, depend on the nature of the membrane lipids. Conversely some proteins have a structural role. For the present purposes, however, the classes of compounds will be considered separately and a synthesis attempted later on.
2.2.1—
Lipids
Of the lipids present in plant cells the various phospholipids, glycolipids and sterols are of the greatest significance in membrane construction. The relative abundance of the components can be quite variable (Table 2.1) depending on the part of the plant analysed, the species and prevailing environmental conditions (see p. 29).
|
2.2.1.1—
Phospholipids
The phospholipid molecule can be separated into a charged or polar 'head' region and an uncharged or non-polar 'tail'. Such a molecule is described as amphipathic, and as we shall see later on, this property is of crucial importance in determining membrane structure (p. 36). Phospholipids are generally thought to be restricted to membranes but the extremely rapid rate at which membranes can be taken apart and re-assembled, as in cell plasmolysis and de-plasmolysis, makes it probable that there are stores of phospholipid within the cell.
Phospholipids are readily extracted from macerated plant tissues by a mixture of chloroform and methanol (2:1) and can be separated by thin layer chromatography using a variety of solvent systems (see Hitchcock & Nichols, 1971, for a review of techniques).
The commonest phospholipids in plant membranes are derivatives of phosphatidic acid (Fig. 2.2); thus, lecithin is the choline ester of phosphatidic acid. Other common derivatives are also shown in Fig. 2.2. Phosphatidic acid (PA) itself is generally said to occur only in minute quantities in membranes or not at all, indeed, its presence in an extract is often taken as an indication of the activity of phospholipase D (Mazliak, 1973). There is a report, however, in which phosphatidic acid is said to be one of the major constituents of the plasmalemma of oat (Avena sativa ) root, (Keenan et al., 1974). Unfortunately,
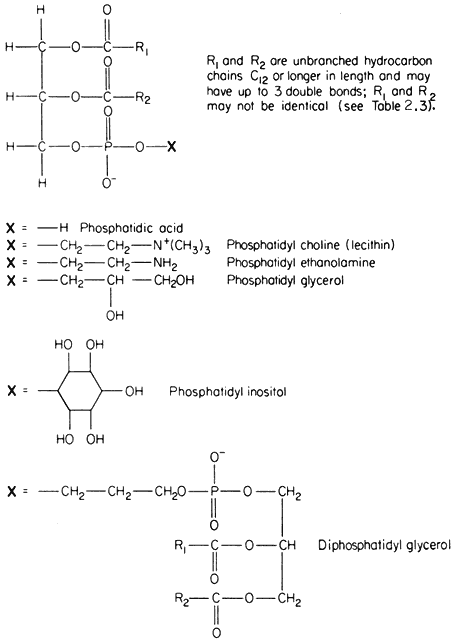
Figure 2.2
Structural formulae of phospholipids commonly found in plants.
detailed analyses of the plasmalemma from other plants are not available for comparison. In passing it might be noted that a great deal remains to be done, firstly in preparing pure sub-cellular fractions of the plasmalemma and of other membranes from plants and subsequently in determining their lipid composition. Table 2.2 presents some of the available information on the distribution of the different types of phospholipid. The information on the composition of the
|
membranes of mitochondria and chloroplasts is the most detailed and reliable since these organelles can be separated with relative ease and high purity during cell fractionation. The predominant phospholipid in a given membrane may be characteristic, e.g. phosphatidylglycerol (PG) is a major component of chloroplast membranes while it is only a minor component of the inner mitochondrial membrane where diphosphatidylglycerol (DPG) is predominant. In general extracts of shoots and roots neither of these phospholipids is as abundant as lecithin (PC) or phosphatidyl ethanolamine (PE). A small quantity of phosphatidyl inositol (PI), usually less than 10% of the total phospholipid, is found in all membranes.
In Fig. 2.2 the exact chain length of the acyl groups R1 and R2 which make up the hydrophobic tail, is not defined precisely. In nature it can vary considerably even in one type of phospholipid from a given tissue. The chain may be made from 12 to 22 carbon atoms and may contain up to three or, rarely, six double bonds. The chain is straight in all eukaryotic organisms and has been found to be branched only in certain bacteria (Asselineau, 1966). Variation in both the length and unsaturation (i.e. the number of double bonds) of the hydrocarbon chain influences its melting point; shorter and unsaturated chains melt at much
lower temperatures than longer and saturated ones. As an example of this consider the effect of double bonds on the melting of free fatty acids containing 18 carbon atoms; the saturated stearic acid (C18:0 ) melts at 69ºC, the monounsaturated oleic acid (C18:1 ) at 5°C and the double unsaturated linoleic acid (C18:2 ) at –12ºC. Organisms which live in warm conditions and warm blooded animals are generally found to have phospholipids with an abundance of fatty acids which tend to be fully saturated (e.g. the thermophilic alga Cyanidium caldarium, see Kleinschmidt & McMahon, 1970). By contrast, organisms which are exposed to lower temperatures have either more unsaturated acids or ones with shorter average chain lengths or a combination of both of these (e.g. in Acholeplasma laidlawii, see Huang et al., 1974) to give phospholipids whose tails remain fluid. The significance of the maintenance of membrane fluidity will become apparent later (p. 41). The process of hardening plants against injury from frost or chilling is accompanied by changes in the degree of unsaturation of the membrane lipids (Wilson & Crawford, 1974).
Table 2.3 shows fatty acid analyses of individual phospholipids extracted from various sources. Bearing in mind that there is a great deal of room for manoeuvre in selecting the fatty acids to suit the prevailing environmental temperature the values for the relative abundance of fatty acids should be considered only as very general guides to the types of acid found in nature. Thus, the predominant fatty acids have even numbers of carbon atoms, the saturated acids found most frequently are palmitic (16:0) and stearic (18:0), and the principal unsaturated acids are linoleic (18:2) and the triply unsaturated linolenic (18:3). The fatty acid composition of lecithin can depend very strongly on its origin. For example, the lecithin in the outer mitochondrial membrane is much richer in palmitic acid (16:0), and perhaps is a less fluid component than in the inner mitochondrial membrane where triply unsaturated linolenic (18:3) is the most abundant fatty acid.
2.2.1.2—
Glycolipids
In several respects the glycolipids resemble phospholipids. The molecule is amphipathic, the polar group being a galactosyl derivative of a diglyceride, the non-polar part of the molecule being a pair of long, straight-chain fatty acids. Glycolipids are unusually rich in the triply unsaturated linolenic acid (C18:3 ) which may make up more than 90% of the fatty acid (Table 2.3).
The two most abundant glycolipids are mono- and di-galactosyl diglyceride, the structural formulae of which are illustrated in Fig. 2.3. They are characteristic of photosynthetic tissues since they are the major lipid component of chloroplast lamellae, largely replacing the phospholipids. Ongun et al., (1968) showed that more than 80% of all of the glycolipid in leaf cells was present in the chloroplasts. The probable orientation of these molecules in the chloroplast lamellae is much like that described for phospholipids (see 2.2.1.1.) with the fatty acid tails inserted into the central region of the membrane with the polar
|
galactosyl groups at the membrane surface protruding into the stroma (Weier & Benson, 1967). Because the fatty acid is so highly unsaturated the membranes of lamellae probably remain fluid even at sub-zero temperatures—thus any photosynthetic reaction, or molecular reorientation, which depends on membrane fluidity may have a wide temperature range in which it can occur.
A sulphur-containing glycolipid is found as a minor component of most membranes. It is known as sulpholipid (Fig. 2.3) and its structure and occurrence in chloroplasts was reported by Benson (1963); the acyl groups are mainly palmitic with a preponderance of linolenic acid, thus resembling the other
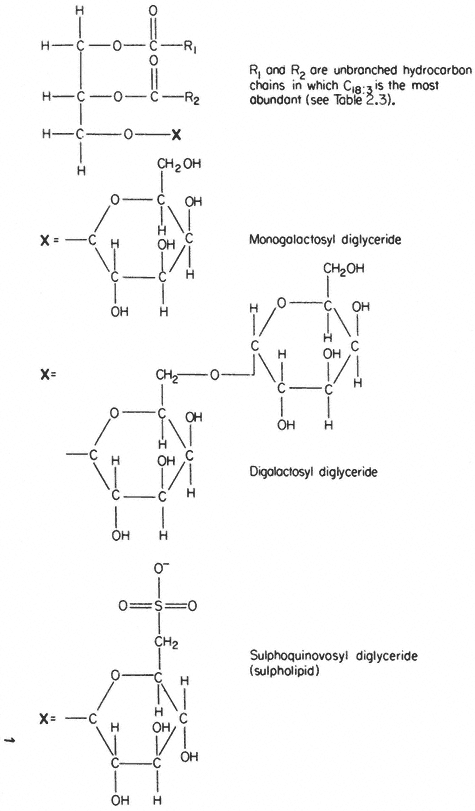
Figure 2.3
Structural formulae of glycolipids commonly found in plants.
galactosyl lipids. Sulpholipid represents only 1% of the total lipid in most tissues and organelles but in chloroplasts it may be as much as 10–15% of the lipid (Ongun & Mudd, 1968).
2.2.1.3—
Sterols
A number of sterols can be extracted from plant tissues and fungi as well as from isolated membrane fractions. The conventional example of a sterol of common biological origin is cholesterol (Fig. 2.4); in practice plant cells contain relatively little of this sterol in comparison with animal cells. Such meagre quantitative data as is available show that sterols having 29 carbon atoms, e.g. b -sitosterol (Fig. 2.4), are the most abundant in higher plants, while in fungi the C-28 sterol, ergosterol (Fig. 2.4) is often dominant. All of these molecules have an extended concertina-like configuration (known as the 'chair' or 'boat'),
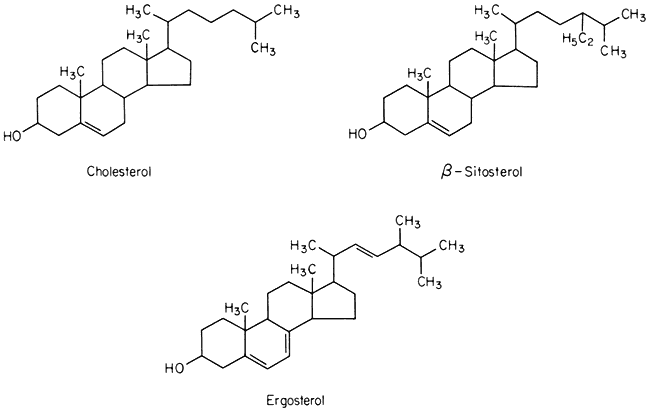
Figure 2.4
Structural formulae of sterols commonly found in biological membranes.
seem metabolically inert and are synthesized and turned over very slowly, especially in comparison with the other lipid components of membranes (Nes, 1974). Their function in membranes is not well understood but it is likely that they have an architectural role concerned with the maintenance of structure or order in the lipid domain. In this respect all of them probably function in the same was as cholesterol (see p. 38) because Butler et al., (1970) found that the structural order of bilayer membranes synthesized from lipids of ox brain tissue was stabilized equally well by cholesterol, b -sitosterol of plant origin and ergosterol. In the plasmalemma of the animal and plant cell there is a much higher proportion of sterols and sterol esters relative to phospholipid than in other
membranes (Table 2.4). It should be noted, however, that the membranes of intracellular organelles contain much more protein than do plasmamembranes (see p. 35). To some extent this protein, much of 2 which is bonded hydrophobically to the lipid, may function in a way similar to sterol in maintaining the structural order of the membrane interior.
|
2.2.2—
Proteins
In many membranes, particularly in those of chloroplasts and mitochondria proteins make up most of the weight. The proteins found are many but as a first step in classifying them integral and peripheral proteins may be distinguished. This classification anticipates the subsequent discussion of membrane structure on page 38 but the terms clearly suggest that proteins in the two classes are associated with other components in the membrane in different ways. The recognition that certain proteins are embedded deeply in the lipid membrane represents a departure from the view, often advanced in earlier texts, that all of the membrane protein is located in the two peripheral bands which stain darkly with osmium and are visualized in the electron microscope (see Fig. 2.1). Whereas some of the protein is certainly located in this way and is probably bonded to the polar regions of the phospholipids electrostatically, it has become apparent that much protein is associated with the non-polar regions of the lipid by hydrophobic bonding. Peripherally located protein can be easily separated by washing with salt solutions or chelating agents but integral proteins are attached very strongly to the membrane and can be removed only after drastic treatment with organic solvents or detergents; even then, the isolated protein usually has some lipid attached to it.
Most of the membrane-bound enzymes, transport proteins (e.g. monovalent cation stimulated ATPase), drug and hormone receptors (in animal cells) and antigenic proteins are integral and are revealed when membranes are split open in freeze-fracturing (see p. 38). In many instances the enzymes arenonfunctional in vitro in the absence of lipid. It is thought that the non-polar parts of the polypeptide chains are associated with the hydrophobic tails of the fatty
acids and, this being so, several types of conformation are possible (see Singer, 1974 for a review). Proteins, like phospholipids, are amphipathic and their polar regions will arrange themselves so that their contacts with the hydrophobic regions of the membrane will be minimized; to ensure this, a protein could be arranged so that its polar, hydrophilic region lies among the phospholipid 'heads', or projects through them into the protein at the membrane periphery (Fig. 2.5).
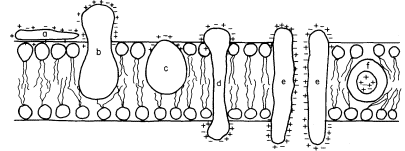
Figure 2.5
Possible orientations of proteins in a membrane. (a) peripherally bound protein
with polar groups all over its surface. (b) and (c) non-polar regions of the protein
bonded hydrophobically to lipid but with different numbers of polar groups. (d) polar
groups at either end of a long molecule with a non-polar central region. (e) a pair of
proteins as in (d) making up a polar pore or channel in the membrane. (f) a hydrophobic
globular protein wholly in the lipid domain—polar groups, if any, directed inwardly.
Long polypeptide chains with charged groups at either end may actually lie across the width of the membrane, or groups of them might lie with polar groups directed inwardly to form a hydrophilic pore across the membrane. An alternative conformation would be provided by the formation of a globular structure in which all of the polar groups would be directed towards the centre of the globule so that a hydrophobic surface would be presented to the lipid. This latter kind of conformation is probably least common.
The peripheral proteins can be attached to the polar groups of either phospholipids or integral proteins; examples which might be taken include cytochrome c which is located on the outer surface of the inner mitochondrial membranes (Schneider et al., 1972; see also Chapter 5), the chromoprotein, phytochrome, which is thought to be attached to the plasmalemma (Marmé et al., 1974; see also Chapter 12) and the sulphate and other ion-binding proteins on the outer surface of bacterial membranes (see Oxender, 1972, for a review).
From a quantitative point of view, certain generalizations about the relative abundance of peripheral and integral proteins can be made. The greater the metabolic activity which centres upon a given membrane system the greater amount of protein integrated into it. Thus, it might be anticipated that chloroplast lamellae and inner mitochondrial membranes would be relatively rich in these proteins, whereas membranes whose role is more concerned with providing
a diffusion barrier, e.g. the plasmalemma and the tonoplast, would be less so; evidence from electron microscopy shows that this is so (see Table 2.5).
2.2.3—
Water
Water is an important, if neglected, constituent of membranes for several reasons. In a general way it determines their basic design since, in its presence, amphipathic lipids assume a bilayered configuration (see p. 36). There are, however, other specific associations of water molecules and membrane components which are not fully understood.
It has been estimated that water of hydration accounts for about 30% of the weight of membranes. Much of this water will almost certainly not be in a liquid state but will exist in ordered layers around the hydrophilic parts of lipids and proteins. Immobilized by hydrogen bonding these water molecules are in a liquid-crystalline condition and cannot be frozen to form ice. Water layers bound at the surface of the membrane have been estimated to have viscosity of 39 times that of pure water and to have a thickness of at least 2.2 nm (Schultz & Asunmaa, 1970). They must contribute to the mechanical stability of membranes and add significantly to their barrier properties to diffusing solutes. Expressing an extreme view, Ling (1973) has proposed that it is not lipid but these polarized multilayers of water which provide the cell with its selective surface barrier.
Hydrophobic bonding between the non-polar regions of lipids and integral proteins (see p. 34) is favoured thermodynamically by the interactions of their polar regions with water (Tait & Franks, 1971).
Much experimental evidence points to the fact that water molecules are not restricted to membrane surfaces but cross the hydrophobic regions in numerous water-filled pores. These pores are thought to conduct water and small solutes (diameter <0.4 nm) to which membranes are highly permeable (see p. 60). Some water lining these pores is fully 'organized' and should, therefore, be regarded as a structural feature but there is indirect evidence to suggest that some of it must be free water in transit.